Which of the following is not part of cell theory? This question delves into the fundamental principles that govern the biological world. Cell theory, a cornerstone of modern biology, elegantly explains the structure, function, and origin of all living things. Understanding its core tenets—that all living organisms are composed of cells, that cells are the basic unit of life, and that all cells arise from pre-existing cells—is crucial.
However, exceptions and nuances exist, prompting critical examination and fostering deeper comprehension of the complexities of life itself. This exploration will clarify common misconceptions and highlight the ongoing evolution of our understanding of cellular biology.
The three main tenets of cell theory—all living things are composed of cells, the cell is the basic unit of life, and all cells come from pre-existing cells—form the bedrock of our understanding of biology. However, certain entities, such as viruses and prions, challenge these tenets, leading to ongoing scientific discussion and refinement of our understanding. This exploration will investigate these challenges, examining the evidence and arguments surrounding the classification of these acellular entities and their implications for the broader definition of life.
Introduction to Cell Theory
Cell theory, a cornerstone of modern biology, provides a fundamental understanding of life’s building blocks. It’s a unifying principle explaining the structure, function, and origin of all living organisms. This section will explore the three main tenets of cell theory, its historical development, and the significant contributions of key scientists.
The Three Tenets of Cell Theory
Cell theory rests on three fundamental principles: All living organisms are composed of one or more cells; the cell is the basic unit of structure and organization in organisms; and all cells arise from pre-existing cells.
- All living organisms are composed of one or more cells: This tenet highlights the universality of the cellular structure of life. Examples include bacteria (prokaryotic cells), single-celled organisms like amoeba (eukaryotic cells), and multicellular organisms like humans (composed of trillions of eukaryotic cells). Viruses, while capable of replication, are not considered living organisms because they lack the cellular structure necessary for independent life.
- The cell is the basic unit of structure and organization in organisms: This emphasizes the cell’s role as the fundamental building block, responsible for carrying out life’s processes. Eukaryotic cells, with their complex organelles, illustrate this principle effectively, with each organelle performing specific tasks. Similarly, prokaryotic cells, despite their simplicity, demonstrate the essential cellular processes necessary for survival within their single-celled structure.
- All cells arise from pre-existing cells: This tenet refutes the idea of spontaneous generation. Cell division, whether mitosis or meiosis, demonstrates this principle, with new cells arising from the division of parent cells. This is evident in the growth and repair of tissues in multicellular organisms and the reproduction of single-celled organisms.
Exceptions to the tenets are rare, primarily concerning the first tenet. Viruses, as mentioned earlier, are acellular and represent an exception. However, their reliance on host cells for replication reinforces the importance of cells in the context of life.
Historical Overview of Cell Theory Development
The development of cell theory was closely tied to advancements in microscopy. The invention and refinement of microscopes allowed scientists to visualize cells and their structures, leading to groundbreaking discoveries.
Date | Scientist(s) | Discovery/Contribution | Significance |
---|---|---|---|
1665 | Robert Hooke | Observed and named “cells” in cork tissue using a compound microscope. | First observation of cells, laying the groundwork for future research. |
Late 17th Century | Antonie van Leeuwenhoek | Observed various single-celled organisms (“animalcules”) using a simple microscope. | Discovery of microorganisms and expanded the understanding of cellular diversity. |
1838 | Matthias Schleiden | Concluded that all plants are made of cells. | Established the cellular basis of plants, contributing to the first two tenets of cell theory. |
1839 | Theodor Schwann | Extended Schleiden’s work to animals, proposing that all animals are also composed of cells. | Formally proposed the first two tenets of cell theory, uniting the plant and animal kingdoms under a common principle. |
1855 | Rudolf Virchow | Proposed “Omnis cellula e cellula” (“All cells come from cells”). | Completed the cell theory by establishing the third tenet, explaining cell reproduction. |
Contributions of Key Scientists
Robert Hooke (1635-1703) observed compartments in cork tissue using his self-designed compound microscope, coining the term “cell.” His detailed sketches of these structures, published inMicrographia* (Hooke, 1665), were crucial in initiating the study of cellular structures. His work, while limited by the technology of his time, provided the first visual evidence of cellular organization.Antonie van Leeuwenhoek (1632-1723), using his single-lens microscopes, discovered a vast world of microscopic organisms, including bacteria and protozoa.
His meticulous observations and detailed descriptions, sent in letters to the Royal Society, revolutionized understanding of microscopic life and significantly broadened the scope of biological investigation. His observations demonstrated the incredible diversity of cellular life.Matthias Schleiden (1804-1881) and Theodor Schwann (1810-1882) are jointly credited with formulating the first two tenets of cell theory. Schleiden’s meticulous study of plant tissues led him to conclude that all plants are composed of cells.
Schwann extended this concept to animals, unifying the plant and animal kingdoms under the common principle of cellular organization. Their collaborative work formed the foundation of modern cell theory.Rudolf Virchow (1821-1902) completed the cell theory with his famous aphorism, “Omnis cellula e cellula” (Virchow, 1858). This statement, emphasizing that all cells arise from pre-existing cells, disproved the long-held belief in spontaneous generation and provided a mechanistic explanation for cell reproduction.
Exploring Common Misconceptions about Cell Theory
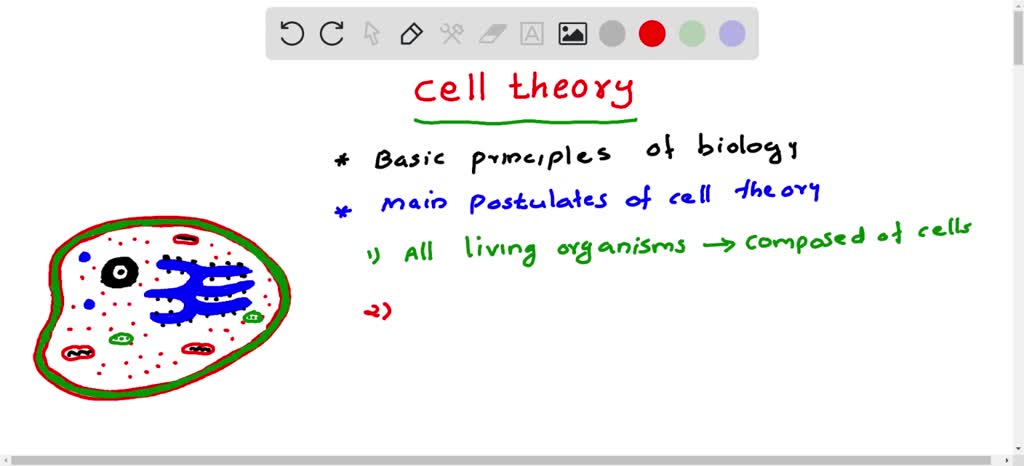
Cell theory, a cornerstone of modern biology, is sometimes misunderstood. While the core principles are relatively straightforward, certain misconceptions persist, leading to inaccurate interpretations of biological processes. Understanding these common misunderstandings is crucial for building a solid foundation in cell biology.
Common Misconceptions about Cell Theory
Several misconceptions about cell theory frequently arise, hindering a proper understanding of its fundamental principles. Addressing these misconceptions directly helps clarify the true nature of cells and their roles in living organisms.
Misconception | Example Statement 1 | Example Statement 2 |
---|---|---|
All cells are identical in structure and function. | “All cells are basically the same; they just have different jobs.” | “Since all life is made of cells, all cells must look and work alike.” |
Spontaneous generation of cells is possible. | “Cells can just appear out of nowhere, especially in simple organisms.” | “New cells can arise from non-living matter under the right conditions.” |
Viruses are considered cells. | “Viruses are tiny cells that infect other cells.” | “Since viruses cause disease, they must be living cells, just very small ones.” |
Explanation of Misconceptions
Misconception 1 Explanation:
- The statement that all cells are identical in structure and function is incorrect. Cells exhibit a remarkable diversity in both structure and function, reflecting their specialized roles within multicellular organisms. For example, nerve cells (neurons) are elongated and possess specialized structures for transmitting electrical signals, while muscle cells are long and fibrous, designed for contraction. Plant cells, unlike animal cells, possess cell walls and chloroplasts.
- This diversity arises from differential gene expression, where different genes are activated in different cell types, leading to the production of specific proteins and the development of unique structures. This specialization allows for the efficient functioning of complex organisms.
- Therefore, the statement ignores the vast array of cell types and their specific adaptations, which are essential for the complexity and diversity of life.
Misconception 2 Explanation:
- The idea of spontaneous generation of cells, also known as abiogenesis in its broader sense, is refuted by the principle that all cells arise from pre-existing cells ( Omnis cellula e cellula). This fundamental tenet of cell theory was established through the work of scientists like Rudolf Virchow.
- The process of cell division, including mitosis and meiosis, ensures the continuity of life by generating new cells from existing ones. There is no known mechanism by which cells can spontaneously arise from non-living matter in modern biological systems.
- The misconception of spontaneous generation reflects a misunderstanding of the fundamental principles of cell reproduction and the continuity of genetic information.
Misconception 3 Explanation:
- Viruses are not considered cells because they lack the fundamental characteristics of living cells. While they can replicate, they require a host cell to do so; they cannot reproduce independently. They lack the necessary cellular machinery for metabolism and protein synthesis.
- Cells are defined by their ability to maintain homeostasis, reproduce independently, and carry out metabolic processes. Viruses, being acellular structures composed of genetic material (DNA or RNA) encased in a protein coat, do not meet these criteria.
- Therefore, classifying viruses as cells is fundamentally inaccurate because they lack the key features that define a cell as a basic unit of life.
Summary of Correct Understandings
All cells share fundamental characteristics, but exhibit significant diversity in structure and function based on their specialized roles. Cells arise only from pre-existing cells through cell division, and viruses, lacking essential cellular components, are not considered cells.
Viruses and Cell Theory
Viruses represent a unique challenge to the tenets of cell theory, primarily because they exist in a gray area between living and non-living entities. While they possess some characteristics of living organisms, their fundamental dependence on host cells for replication and their lack of independent metabolism fundamentally contradict the cell theory’s assertion that all living organisms are composed of cells and exhibit self-sufficiency.
This section will explore the ways in which viruses challenge cell theory and the arguments surrounding their classification.
Acellular Nature of Viruses
Viruses are acellular, meaning they lack the fundamental structural components of cells. Unlike cells, they do not possess a cytoplasm, ribosomes, or other membrane-bound organelles. Instead, a virus consists essentially of genetic material (either DNA or RNA) enclosed within a protein coat called a capsid, and sometimes an outer lipid envelope derived from the host cell membrane. This acellular nature dictates their complete reliance on host cells for replication.
For example, the influenza virus is an enveloped virus with a single-stranded RNA genome, lacking the machinery to independently replicate its genetic material or synthesize proteins. Similarly, bacteriophages, viruses that infect bacteria, possess a capsid and a DNA or RNA genome, but lack the cellular components needed for independent metabolism or reproduction. The acellular nature of viruses necessitates their parasitic lifestyle, highlighting a direct contradiction to cell theory.
Metabolic Dependence of Viruses on Host Cells
Viruses are entirely metabolically dependent on their host cells. They lack the enzymes and machinery necessary for energy production, protein synthesis, or other metabolic processes. To replicate, viruses must hijack the host cell’s machinery, essentially forcing the cell to produce viral components. For instance, HIV, the human immunodeficiency virus, utilizes the reverse transcriptase enzyme of the host cell to convert its RNA genome into DNA, which is then integrated into the host cell’s genome.
This allows the virus to utilize the host cell’s resources to replicate its genetic material and produce viral proteins. The complete reliance on host cells for all metabolic functions directly contradicts the cell theory’s implication of self-sufficiency in living organisms.
Genetic Material of Viruses
Viral genetic material varies significantly compared to cellular organisms. While cellular organisms typically possess double-stranded DNA as their genetic material, viruses can have either DNA or RNA, and this genetic material can be single-stranded or double-stranded. This diversity in genetic material contributes to their ability to evade host immune systems and adapt to new hosts. For example, the rapid mutation rate of RNA viruses like influenza makes it difficult for the immune system to develop long-lasting immunity, leading to the need for annual flu vaccines.
The variations in viral genetic material also contribute to their ability to infect a wide range of hosts, highlighting their unique evolutionary strategies.
Structural Comparison of Cells and Viruses
The following table compares the structures of a typical prokaryotic cell (e.g., bacterium), a typical eukaryotic cell (e.g., animal cell), and a bacteriophage (a virus that infects bacteria) to illustrate the fundamental differences.
Feature | Prokaryotic Cell | Eukaryotic Cell | Bacteriophage |
---|---|---|---|
Cell Membrane | Present | Present | Absent |
Genetic Material | Circular DNA | Linear DNA | DNA or RNA (single or double-stranded) |
Ribosomes | Present (70S) | Present (80S) | Absent |
Cytoplasm | Present | Present | Absent |
Capsid | Absent | Absent | Present |
Viral Reproductive Strategies
Viruses employ two primary reproductive strategies: the lytic cycle and the lysogenic cycle. In the lytic cycle, the virus quickly replicates its genetic material and produces new virions, ultimately lysing (breaking open) the host cell and releasing the progeny viruses. In the lysogenic cycle, the viral genome integrates into the host cell’s genome, remaining dormant until environmental triggers induce the lytic cycle.
These strategies contrast sharply with the reproductive mechanisms of cellular organisms, such as binary fission (prokaryotes) and mitosis/meiosis (eukaryotes), which involve the controlled division of the cell itself.
Viral Assembly Process
Viral assembly is a complex process that relies heavily on the host cell’s machinery. Following the replication of viral genetic material and the synthesis of viral proteins, these components self-assemble into new virions. The specific steps vary depending on the virus, but generally involve the packaging of the viral genome into the capsid and, in enveloped viruses, the acquisition of a lipid envelope from the host cell membrane.
Host cell proteins often play a crucial role in this assembly process. For instance, the influenza virus uses host cell enzymes to cleave its hemagglutinin protein, a crucial step in viral release.
Metabolic Inactivity of Viruses
The lack of independent metabolism is a central argument against classifying viruses as living organisms. Viruses cannot produce their own energy or synthesize their own building blocks; they rely entirely on the host cell for these functions. Unlike cellular organisms that perform various metabolic processes, viruses are metabolically inert outside of a host cell. This complete dependence on a host cell for all metabolic needs is a key characteristic distinguishing viruses from living organisms.
Absence of Growth and Development in Viruses
Viruses do not exhibit growth and development in the same way as cellular organisms. While viral replication results in an increase in the number of virions, this is not considered growth in the same sense as the increase in cell size and complexity observed in cellular organisms. Viruses do not undergo developmental stages or exhibit any increase in complexity during their life cycle.
Their replication is a purely multiplicative process, lacking the intricate developmental pathways seen in living organisms.
Limited Response to Stimuli in Viruses
Viruses exhibit a very limited response to external stimuli compared to living cells. They do not actively seek out hosts or respond to changes in their environment in the same way that living organisms do. Their interaction with the environment is largely passive; they rely on chance encounters with susceptible host cells. This limited responsiveness, contrasting with the complex signaling pathways and adaptive responses of living cells, further supports the argument against classifying viruses as living organisms.
Further Considerations on Viruses and the Definition of Life
The ambiguous nature of viruses, possessing some characteristics of living organisms while lacking others, challenges our understanding of life itself. Their ability to participate in horizontal gene transfer, where genetic material is transferred between organisms without reproduction, significantly impacts the evolution of other organisms. This process contributes to the evolution of both prokaryotes and eukaryotes, blurring the lines further between what constitutes a living organism and the role of viruses in the grand scheme of life’s origins and evolution.
Prions and Cell Theory
Prions, unlike viruses or bacteria, challenge the traditional tenets of cell theory because they are infectious agents that lack nucleic acids (DNA or RNA), the fundamental genetic material considered essential for replication and the transmission of heritable information, a cornerstone of cell theory. Understanding prions and their unique mechanism of action is crucial for clarifying the limitations of cell theory in encompassing all forms of biological agents.Prions are misfolded proteins that can induce other properly folded proteins of the same type to also misfold, creating a chain reaction that leads to the accumulation of abnormal prion proteins in the brain and other tissues.
This accumulation disrupts cellular function, leading to neurodegenerative diseases like Creutzfeldt-Jakob disease (CJD) in humans, bovine spongiform encephalopathy (BSE, or “mad cow disease”) in cattle, and scrapie in sheep. The misfolded prion protein is designated PrP Sc, while the normal cellular form is PrP C. The conversion of PrP C to PrP Sc is the key event in prion disease pathogenesis.
This process does not involve the replication of genetic material, making it fundamentally different from viral infections.
Prion Structure and Replication
Prions are composed solely of protein; they lack any genetic material. Their replication mechanism is unique and involves the templating of misfolded proteins. The abnormal PrP Sc protein acts as a template, inducing the misfolding of normal PrP C proteins into the abnormal conformation. This self-perpetuating process leads to the accumulation of misfolded proteins, causing cellular damage and ultimately, disease.
This mechanism differs significantly from viruses, which require their own genetic material to replicate and hijack host cellular machinery.
Comparison of Viruses, Prions, and Cells
The following table highlights key differences between viruses, prions, and cells, emphasizing aspects relevant to cell theory.
Feature | Virus | Prion | Cell |
---|---|---|---|
Genetic Material | DNA or RNA | None | DNA |
Structure | Nucleic acid enclosed in a protein coat (capsid); some have an envelope | Misfolded protein | Membrane-bound structure containing organelles and genetic material |
Replication | Requires host cell machinery | Template-assisted misfolding of proteins | DNA replication and cell division |
Infectivity | Infectious; can spread from cell to cell | Infectious; can spread from cell to cell | Not inherently infectious in the same way as viruses or prions |
Cellular Function Disruption | Disrupts host cell function through replication and resource depletion | Disrupts cellular function through protein misfolding and aggregation | Carries out a wide range of metabolic functions |
Exceptions and Limitations
While cell theory forms the bedrock of modern biology, recent scientific discoveries have revealed exceptions and limitations to its universally accepted tenets. These exceptions don’t invalidate the theory but rather highlight its complexity and the need for continuous refinement in light of new evidence. Understanding these limitations expands our comprehension of the diverse forms and functionalities of life.
The classical cell theory posits that all living organisms are composed of cells, that cells are the basic unit of life, and that all cells arise from pre-existing cells. However, certain biological entities challenge these postulates, forcing us to consider nuances and refine our understanding.
Organelles and Cell Theory
Certain cellular structures, while essential for cellular function, present a subtle challenge to the notion of the cell as the fundamental unit of life. Mitochondria and chloroplasts, for example, possess their own DNA and ribosomes, suggesting an independent evolutionary origin. The endosymbiotic theory proposes that these organelles were once free-living prokaryotes that established a symbiotic relationship with eukaryotic cells.
This challenges the strict definition of the cell as the smallest unit of life, as these organelles exhibit some degree of autonomy within the larger cellular context. The intricate interplay between the host cell and its organelles illustrates the complex hierarchical organization of life, exceeding a simple, singular cell-based definition.
Viruses and the Origin of Cells, Which of the following is not part of cell theory
Viruses, acellular entities composed of genetic material enclosed in a protein coat, represent a significant exception to the cell theory. They lack the cellular machinery for independent replication and metabolism, requiring a host cell to reproduce. This obligate intracellular parasitism blurs the lines between living and non-living entities. While not cells themselves, viruses play a crucial role in evolution, potentially contributing to the genetic diversity of cellular life.
The origin of viruses remains a topic of ongoing research, with some hypotheses suggesting they evolved from cellular components or even predate the origin of cells. Understanding viral evolution is essential for comprehending the origins and diversification of life itself.
Prions and the Central Dogma
Prions, infectious misfolded proteins, challenge the central dogma of molecular biology, which states that information flows from DNA to RNA to protein. Prions can induce conformational changes in normal proteins, leading to the propagation of the misfolded state. This process, devoid of nucleic acid involvement, demonstrates a form of biological information transfer that bypasses the conventional DNA-RNA-protein pathway. The implications for our understanding of heredity and disease are significant, highlighting the limitations of traditional models of biological information transfer.
The ability of a protein alone to transmit heritable information, as seen in prion diseases, adds another layer of complexity to our understanding of life’s fundamental processes.
Synthetic Cells and Cell Theory
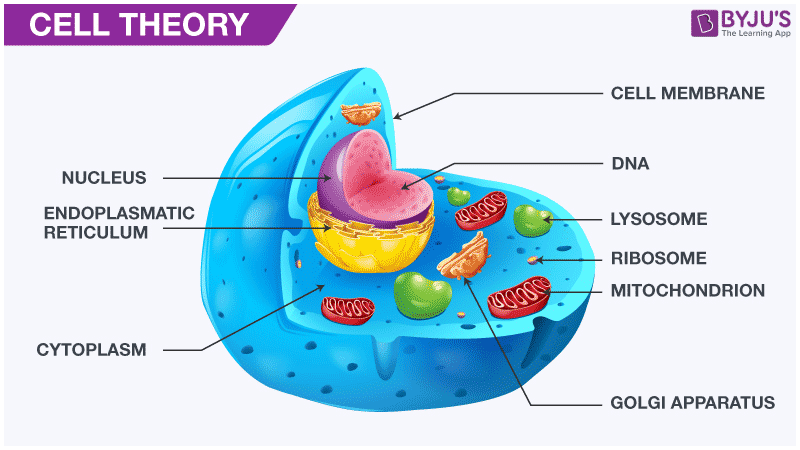
The creation of synthetic cells represents a landmark achievement in biological engineering, profoundly impacting our understanding of life and challenging the established tenets of cell theory. This section delves into the process of synthetic cell creation, its implications for cell theory, ethical considerations, and potential future applications.
Synthetic Cell Creation Process
Creating a synthetic cell involves a multi-step process. First, a minimal genome, containing only the essential genes for life, is selected. This genome is then chemically synthesized, often using automated DNA synthesis techniques. The synthetic genome is subsequently encapsulated within a cell-like structure, typically a liposome or a pre-existing cell’s cytoplasm that has had its own genome removed. Finally, the encapsulated genome is reconstituted, resulting in a functional, albeit simplified, cell.
The following flowchart illustrates this process:
Flowchart: Synthetic Cell Creation
Step 1: Minimal Genome Selection → Step 2: Synthetic Genome Construction → Step 3: Genome Encapsulation → Step 4: Cellular Reconstitution → Synthetic Cell
Impact on Cell Theory
The creation of synthetic cells directly challenges and supports aspects of cell theory. Classical cell theory posits that all cells arise from pre-existing cells (*Omnis cellula e cellula*). Synthetic cell research, however, demonstrates the possibility of creating a functional cell from non-cellular components, albeit starting with pre-existing cellular machinery in some cases. The definition of life itself is also debated in light of synthetic cells; while lacking the complexity of natural cells, they exhibit key characteristics of life, such as metabolism and growth (in some cases).
The role of genetic information remains central, highlighting the importance of DNA in directing cellular processes, even in simplified synthetic systems.
Aspect of Cell Theory | Classical Cell Theory Prediction | Observation from Synthetic Cell Research |
---|---|---|
Origin of Cells (*Omnis cellula e cellula*) | All cells arise from pre-existing cells through cell division. | Challenges this tenet by demonstrating the possibility of creating a functional cell from non-cellular components, though often relying on pre-existing cellular components for initial construction. |
Definition of Life | Life is characterized by complex organization, metabolism, growth, reproduction, and response to stimuli. | Synthetic cells, while simpler than natural cells, exhibit some of these characteristics, blurring the lines of the definition of life. |
Role of Genetic Information | Genetic information (DNA) is essential for cellular function. | Confirmed by the necessity of a functional genome in creating a synthetic cell. |
Naturally Occurring vs. Synthetic Cells
A comparison of naturally occurring cells and synthetic cells highlights the significant differences in their complexity and capabilities.
Feature | Naturally Occurring Cell (e.g.,E. coli*) | Synthetic Cell (e.g., JCVI-syn1.0) |
---|---|---|
Genome Size and Complexity | Large and complex genome with thousands of genes. | Minimal genome with a significantly reduced number of genes. |
Metabolic Capabilities | Wide range of metabolic pathways. | Limited metabolic capabilities, dependent on a defined nutrient medium. |
Reproducibility | Self-replicating. | Initially not self-replicating; later versions have shown limited self-replication capabilities. |
Cellular Structure and Organization | Complex cellular structure with various organelles. | Simpler cellular structure, often lacking many organelles. |
Ethical Considerations
The creation of synthetic cells raises several ethical concerns. Careful consideration of these issues is crucial for responsible development and application of this technology.
- Biosecurity concerns: The potential for misuse of synthetic cells to create bioweapons or harmful pathogens needs to be addressed through stringent regulations and oversight.
- Environmental impact: The release of synthetic cells into the environment could have unforeseen ecological consequences. Risk assessment and mitigation strategies are necessary.
- Potential for misuse: The technology could be misused for malicious purposes, such as creating designer pathogens or disrupting ecosystems.
Future Applications
Synthetic cells hold immense potential across various fields.
- Medicine: Development of novel therapeutics, targeted drug delivery systems, and biocompatible materials.
- Biomanufacturing: Production of valuable biomolecules, such as pharmaceuticals and biofuels, in a sustainable and cost-effective manner.
- Environmental remediation: Development of synthetic cells capable of degrading pollutants and cleaning up contaminated environments.
Specific Example: JCVI-syn1.0
JCVI-syn1.0, the first self-replicating synthetic cell, was created by transplanting a synthetically constructed genome into a recipient cell. The source organism was
- Mycoplasma mycoides*, and the genome was synthesized using a combination of automated DNA synthesis and gene assembly techniques. The resulting synthetic cell exhibited similar characteristics to the original
- M. mycoides*, demonstrating the feasibility of creating a functional cell from a chemically synthesized genome.
“We have created a new, self-replicating cell whose genome is entirely computer-designed and chemically synthesized.”
Venter et al., Science, 2010
Limitations of Current Synthetic Cell Technology
Despite significant progress, limitations remain in creating fully functional and self-replicating synthetic cells.
- Precise control over genome assembly and function: Challenges remain in precisely controlling the assembly and expression of complex genomes.
- Understanding minimal gene sets: A complete understanding of the minimal set of genes required for cellular life is still lacking.
- Encapsulation and reconstitution efficiency: Efficient and reliable methods for encapsulating and reconstituting synthetic genomes are needed.
- Long-term stability and viability: Maintaining the long-term stability and viability of synthetic cells remains a challenge.
The Role of Cell Organelles: Which Of The Following Is Not Part Of Cell Theory
Cell organelles are specialized subunits within cells that carry out specific functions necessary for cell survival and overall organismal function. Their coordinated actions ensure the cell’s efficient operation, encompassing processes from energy production to waste disposal and protein synthesis. Understanding the roles of individual organelles and their interactions is crucial for comprehending cellular processes and the implications of their malfunction.
Functions of Major Cell Organelles
The major cell organelles each play a distinct yet interconnected role in maintaining cellular homeostasis. The nucleus houses the genetic material, directing cellular activities; mitochondria generate energy; ribosomes synthesize proteins; the endoplasmic reticulum modifies and transports proteins; the Golgi apparatus processes and packages proteins; lysosomes break down waste; vacuoles store materials; and chloroplasts (in plants) conduct photosynthesis.
Determining which statement isn’t part of cell theory requires a clear understanding of its foundational principles. This contrasts sharply with the complexities explored in epistemology, such as what is the class theory of knowledge about , which delves into the nature of knowledge itself. Therefore, carefully examining each proposed component against the established tenets of cell theory is crucial for accurate identification.
- Nucleus: The control center of the cell, housing the DNA and directing gene expression. Examples include DNA replication, transcription (RNA synthesis), and regulation of gene expression controlling cellular processes like cell division and differentiation.
- Mitochondria: The powerhouses of the cell, responsible for cellular respiration and ATP production. Examples include oxidative phosphorylation, the citric acid cycle (Krebs cycle), and beta-oxidation of fatty acids.
- Ribosomes: Sites of protein synthesis, translating mRNA into polypeptide chains. Examples include synthesis of structural proteins (like collagen), enzymes (like DNA polymerase), and hormones (like insulin).
- Endoplasmic Reticulum (ER): A network of membranes involved in protein and lipid synthesis and transport. Examples include protein folding and modification within the rough ER, lipid synthesis in the smooth ER, and calcium ion storage.
- Golgi Apparatus: Processes, modifies, and packages proteins and lipids for secretion or transport within the cell. Examples include glycosylation of proteins, sorting proteins for delivery to different cellular locations, and the formation of lysosomes.
- Lysosomes: Contain digestive enzymes that break down waste materials, cellular debris, and pathogens. Examples include autophagy (recycling of cellular components), phagocytosis (engulfing pathogens), and apoptosis (programmed cell death).
- Vacuoles: Storage compartments for water, nutrients, and waste products; larger and more prominent in plant cells. Examples include maintaining turgor pressure in plant cells, storing pigments, and sequestering toxic substances.
- Chloroplasts (Plant Cells): Sites of photosynthesis, converting light energy into chemical energy in the form of glucose. Examples include the light-dependent reactions, the Calvin cycle (carbon fixation), and starch storage.
Animal and Plant Cell Diagram Descriptions
Organelle interactions are vital for cellular function. The following descriptions illustrate these interactions in animal and plant cells.> Animal Cell Diagram Description: Imagine a roughly spherical cell. The nucleus, a large, round structure, is centrally located. Scattered throughout the cytoplasm are numerous smaller, oval-shaped mitochondria. The endoplasmic reticulum (ER) forms a network of interconnected membranes extending throughout the cytoplasm, with ribosomes attached to the rough ER.
The Golgi apparatus, a stack of flattened sacs, is typically located near the nucleus. Lysosomes are small, membrane-bound vesicles scattered throughout the cytoplasm.>
Interactions
The nucleus directs protein synthesis by producing mRNA, which is transported to ribosomes on the rough ER. Proteins synthesized on the rough ER are then transported to the Golgi apparatus for modification and packaging. Mitochondria provide energy (ATP) for all cellular processes, including protein synthesis and transport. Lysosomes break down waste products from various cellular processes.> Plant Cell Diagram Description: Similar to the animal cell, but with key differences.
A large central vacuole dominates the cell’s interior, pushing the nucleus and other organelles towards the periphery. Chloroplasts, oval-shaped organelles containing chlorophyll, are scattered throughout the cytoplasm. A rigid cell wall surrounds the cell membrane. The other organelles (nucleus, mitochondria, ER, Golgi, and ribosomes) are present but occupy a smaller relative volume compared to the central vacuole.>
Interactions
Similar interactions occur as in the animal cell, with the addition of chloroplasts generating glucose through photosynthesis, which is then used by the mitochondria for energy production. The central vacuole maintains turgor pressure, supporting the cell’s structure. The cell wall provides structural support and protection. The differences lie in the presence of a large central vacuole, chloroplasts, and a cell wall in the plant cell, absent in the animal cell.
Malfunction of Specific Organelles and Cell Theory
Malfunctions in specific organelles can significantly impact cellular function and provide evidence supporting or contradicting aspects of cell theory.
Organelle | Malfunction | Effect on Cell Function | Support/Contradiction of Cell Theory Aspect | Explanation |
---|---|---|---|---|
Ribosomes | Mutations in ribosomal RNA genes leading to impaired ribosomal function | Reduced or absent protein synthesis; inability to produce essential proteins | Supports: All cells require protein synthesis for basic functions. | The inability to produce proteins directly affects the cell’s ability to function, demonstrating the fundamental role of protein synthesis in all living cells, a core tenet of cell theory. |
Mitochondria | Mitochondrial DNA mutations leading to reduced ATP production | Decreased energy levels; impaired cellular processes; potential cell death | Supports: Cells require energy for life processes. | Reduced ATP production severely limits the cell’s capacity to perform its functions, highlighting the dependence of all cells on energy production for survival, directly supporting the cell theory. |
Lysosomes | Lysosomal enzyme deficiency (e.g., in Tay-Sachs disease) | Accumulation of undigested materials within the cell; cellular dysfunction | Supports: Cells require mechanisms for waste removal. | The buildup of waste products directly damages cellular components and impairs normal function, highlighting the necessity of efficient waste removal systems in all cells. This supports the aspect of cell theory stating that cells maintain homeostasis. |
Comparison of Prokaryotic and Eukaryotic Cells
Prokaryotic and eukaryotic cells differ significantly in their structure and organization, reflecting differences in their cellular processes.
Feature | Prokaryotic Cell | Eukaryotic Cell |
---|---|---|
Nucleus | Absent; DNA is located in the nucleoid region | Present; DNA is enclosed within a membrane-bound nucleus |
Mitochondria | Absent; energy production occurs in the cytoplasm | Present; responsible for cellular respiration |
Ribosomes | Present; smaller than eukaryotic ribosomes (70S vs 80S) | Present; larger ribosomes (80S) |
Cell Wall | Present; usually composed of peptidoglycan | Present in plant cells (cellulose); absent in animal cells |
Membrane-bound organelles | Absent | Present (e.g., nucleus, mitochondria, ER, Golgi, lysosomes) |
Size | Generally smaller (1-5 μm) | Generally larger (10-100 μm) |
Disease Resulting from Organelle Malfunction
Tay-Sachs disease is a lysosomal storage disorder resulting from a deficiency in the enzyme hexosaminidase A. This enzyme is crucial for breaking down a specific type of lipid called GM2 ganglioside. The deficiency leads to the accumulation of GM2 ganglioside in the brain and other tissues, causing progressive neurological damage, developmental delays, and ultimately death. The affected organelle is the lysosome.
Cell Size and Cell Theory
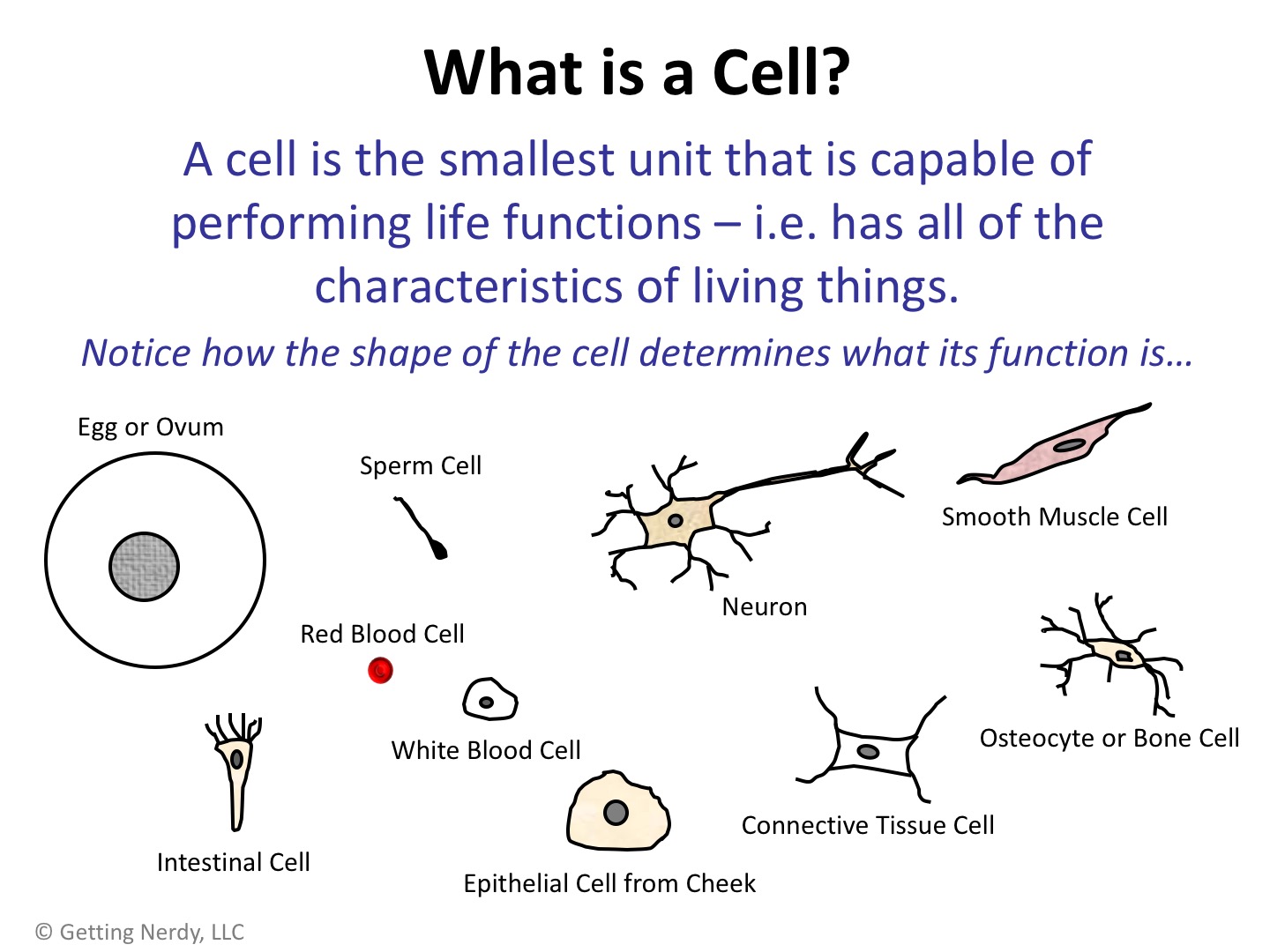
Cell size is a critical factor influencing cell function and overall organismal biology. The limitations imposed by cell size directly impact the principles of cell theory, particularly the concepts of cell function and reproduction. Understanding the relationship between size and function reveals fundamental constraints and remarkable adaptations in the biological world.The surface area-to-volume ratio is a key determinant of a cell’s ability to efficiently exchange materials with its environment.
As a cell grows larger, its volume increases at a faster rate than its surface area. This means that a larger cell has relatively less surface area available for nutrient uptake, waste removal, and gas exchange. This limitation directly affects the cell’s metabolic processes and ultimately restricts its size. Smaller cells, with their higher surface area-to-volume ratio, are more efficient at transporting substances across their membranes.
This efficiency is crucial for maintaining cellular homeostasis and supporting the rapid rates of metabolic activity often observed in smaller cells.
Surface Area-to-Volume Ratio and Cellular Function
The surface area-to-volume ratio dictates the efficiency of nutrient uptake and waste removal. A high ratio, characteristic of smaller cells, facilitates rapid exchange. Conversely, a low ratio, found in larger cells, limits this exchange, impacting metabolic rate and overall cell function. For example, a spherical cell with a radius of 1 micrometer has a surface area of approximately 12 square micrometers and a volume of approximately 4 cubic micrometers, resulting in a high surface area-to-volume ratio.
In contrast, a spherical cell with a radius of 10 micrometers has a surface area of approximately 1256 square micrometers and a volume of approximately 4189 cubic micrometers, resulting in a significantly lower surface area-to-volume ratio. This difference in ratio directly impacts the cell’s ability to sustain its metabolic processes. The smaller cell is far more efficient at nutrient and waste exchange.
Examples of Cells Deviating from Average Size and Their Adaptations
Many cells deviate significantly from the average cell size, showcasing remarkable adaptations to overcome the limitations of size. Nerve cells, for instance, are exceptionally long and thin, maximizing their surface area for signal transmission over long distances. Their elongated shape allows for efficient conduction of nerve impulses along their axons. This specialized morphology effectively circumvents the limitations imposed by a simple increase in cell volume.
Another example is the giant amoeba, which can reach macroscopic sizes. To compensate for their low surface area-to-volume ratio, these amoebas utilize specialized mechanisms for intracellular transport and efficient waste removal. These mechanisms are crucial for maintaining cellular homeostasis despite their large size. Furthermore, the elongated shape of some bacterial cells likeE. coli* allows for a greater surface area for nutrient absorption compared to a spherical cell of the same volume.
This adaptation is crucial for their rapid growth and reproduction.
Cell Reproduction and Cell Theory
Cell reproduction, encompassing both mitosis and meiosis, is fundamentally linked to the tenets of cell theory. The processes by which cells divide and create new cells directly support the idea that all cells arise from pre-existing cells. Understanding these reproductive mechanisms is crucial to fully grasping the implications of cell theory and its exceptions.Cell reproduction mechanisms directly support the central dogma of cell theory, namely that all cells come from pre-existing cells.
This principle is demonstrably true through observation of both mitosis and meiosis, the two primary methods of cell division. These processes ensure the faithful transmission of genetic material, maintaining the continuity of life from one generation to the next. However, the specifics of these processes also reveal some interesting nuances and challenges to the strict interpretation of cell theory.
Mitosis and Meiosis: Mechanisms of Cell Division
Mitosis is a type of cell division that results in two daughter cells each having the same number and kind of chromosomes as the parent nucleus, typical of ordinary tissue growth. It is a crucial process for growth, repair, and asexual reproduction in many organisms. Meiosis, on the other hand, is a type of cell division that reduces the number of chromosomes in the parent cell by half and produces four gamete cells.
This process is essential for sexual reproduction, creating genetic diversity within a population. Both processes involve a complex series of steps, including DNA replication, chromosome condensation, and spindle formation, ensuring accurate segregation of chromosomes into daughter cells. The precise regulation of these processes is vital for maintaining genomic stability and preventing errors that could lead to cell dysfunction or disease.
How Cell Division Supports Cell Theory
The observation of mitosis and meiosis directly supports the central tenet of cell theory: Omnis cellula e cellula – all cells arise from pre-existing cells. The meticulous replication and division of genetic material during these processes demonstrate that new cells are not spontaneously generated but are instead products of the division of existing cells. The continuity of genetic information across generations, ensured by the fidelity of these processes, provides strong evidence for this principle.
Furthermore, the study of cell division has allowed scientists to understand how errors in these processes can lead to genetic mutations and diseases, further solidifying the link between cell reproduction and the overall principles of cell theory.
Exceptions and Challenges to Cell Theory Related to Cell Reproduction
While cell division largely supports cell theory, some exceptions and challenges exist. The origin of the very first cells remains a subject of ongoing research and debate, as the spontaneous generation of life from non-living matter is a concept that predates the current understanding of cell theory. Additionally, the existence of organelles with their own DNA, such as mitochondria and chloroplasts, presents a slight complication.
The endosymbiotic theory suggests these organelles were once independent prokaryotic cells that were engulfed by eukaryotic cells, a process that doesn’t strictly adhere to the ‘all cells from pre-existing cells’ rule in its entirety. However, even this theory supports the idea of cellular origins and evolution, fitting within the broader context of cell theory. The study of synthetic cells, created artificially in laboratories, also presents an interesting case study in challenging and extending the established boundaries of cell theory.
Acellular Life Forms and Cell Theory
Cell theory, a cornerstone of modern biology, posits that all living organisms are composed of cells and that all cells arise from pre-existing cells. However, the existence of certain acellular entities challenges this seemingly absolute statement, prompting a nuanced understanding of the theory’s scope and limitations. This section explores acellular life forms and their relationship to cell theory.Acellular life forms are biological entities that lack the typical cellular structure found in all other living organisms.
Unlike cellular organisms, which are characterized by membrane-bound organelles, a nucleus containing genetic material, and a complex metabolic machinery, acellular entities are simpler in their organization and often rely on a host cell for replication and metabolic processes. The most prominent examples are viruses and prions, both of which raise important questions about the definition of life itself and the universality of cell theory.
Characteristics of Acellular Life Forms
Acellular life forms, such as viruses and prions, significantly differ from cellular life in their structure and life cycle. They are not considered truly “alive” in the same way as cellular organisms because they lack the capacity for independent metabolism and reproduction. Instead, they are obligate intracellular parasites, meaning they require a host cell to replicate and carry out their life functions.
This dependence on host cells is a key distinction between acellular and cellular life forms. Their genetic material, which may be DNA or RNA, is not enclosed within a nucleus but rather exists within a protein coat or capsid (in the case of viruses). Prions, on the other hand, consist solely of misfolded proteins.
Comparison of Acellular and Cellular Life Forms
The following points highlight the key differences between acellular and cellular life forms in the context of cell theory:
- Cellular Structure: Cellular organisms possess a defined cellular structure with membrane-bound organelles, including a nucleus containing genetic material. Acellular organisms lack this organized cellular structure.
- Metabolism: Cellular organisms carry out independent metabolism, producing energy and synthesizing necessary molecules. Acellular organisms lack independent metabolic capabilities and rely on the host cell’s metabolic machinery.
- Reproduction: Cellular organisms reproduce independently through processes like mitosis or meiosis. Acellular organisms are obligate intracellular parasites and require a host cell for replication.
- Genetic Material: Cellular organisms possess their genetic material (DNA) within a membrane-bound nucleus. Acellular organisms may possess DNA or RNA, but it is not enclosed within a nucleus.
- Growth and Development: Cellular organisms exhibit growth and development throughout their life cycle. Acellular organisms do not exhibit growth and development in the same way; they assemble within the host cell.
Implications for Cell Theory
The existence of acellular life forms necessitates a refinement of cell theory. While the core principle—that cells are the fundamental units of life—remains valid for cellular organisms, it needs to acknowledge the existence of these entities that do not conform to the traditional definition of a cell. The cell theory is not invalidated, but rather its scope is clarified to specifically address cellular life.
The study of acellular life forms provides valuable insights into the origins of life, the evolution of parasitism, and the fundamental principles of biology.
The Origin of Cells
The origin of cells, the fundamental units of life, remains a central question in biology. Understanding this origin is crucial for comprehending the evolution of life on Earth and the very nature of life itself. Several hypotheses attempt to explain how the first cells arose from simpler, non-living matter, a process known as abiogenesis. These hypotheses, while differing in detail, all grapple with the challenge of explaining the transition from inorganic molecules to self-replicating, membrane-bound entities.The origin of cells is intrinsically linked to the tenets of cell theory, which states that all living organisms are composed of cells, and that all cells arise from pre-existing cells.
The origin of the first cell, therefore, represents a critical exception to this latter tenet, posing a significant challenge to our understanding of cellular life’s history. The evolution of cells from simpler structures involved a series of complex steps, requiring the emergence of self-replication, metabolism, and compartmentalization.
Hypotheses on the Origin of Cells
Several hypotheses attempt to explain the transition from non-living matter to the first cells. These hypotheses often focus on different aspects of the problem, such as the formation of self-replicating molecules, the development of metabolic pathways, or the creation of membrane-bound compartments. One prominent hypothesis suggests that life originated in hydrothermal vents, where chemical gradients could have provided the energy needed for early metabolic processes.
Another suggests that life arose in shallow pools of water, where organic molecules could have concentrated and interacted. These environments offered protection from harmful UV radiation and provided a stable environment for the development of early life. A third hypothesis proposes that life originated in clay minerals, which could have acted as catalysts for the formation of complex organic molecules.
The specifics of each hypothesis are still under investigation, and it is likely that the origin of life was a complex process involving multiple factors.
The Role of RNA in Early Cells
The RNA world hypothesis proposes that RNA, rather than DNA, was the primary genetic material in early cells. RNA possesses both catalytic and informational properties, making it a plausible candidate for the first self-replicating molecule. Ribozymes, catalytic RNA molecules, have been shown to catalyze a variety of reactions, including the synthesis of other RNA molecules. This suggests that early RNA molecules could have replicated themselves and catalyzed the reactions necessary for metabolism.
The transition from an RNA world to a DNA world is thought to have occurred later, as DNA is a more stable molecule and better suited for long-term storage of genetic information.
The Evolution of Cellular Structures
The evolution of cells from simpler structures involved the development of key features such as membranes, ribosomes, and a genetic system. The formation of lipid membranes is thought to have been a crucial step, as membranes provide a compartmentalized environment that allows for the concentration of reactants and the separation of internal and external environments. The evolution of ribosomes, the cellular machinery responsible for protein synthesis, is another key event.
Ribosomes are complex molecular machines, and their evolution likely involved the gradual assembly of ribosomal RNA and proteins. The development of a genetic system, whether based on RNA or DNA, was essential for the inheritance of traits and the evolution of cells. This involved the development of mechanisms for DNA replication, transcription, and translation.
The Endosymbiotic Theory
The endosymbiotic theory explains the origin of mitochondria and chloroplasts, organelles found in eukaryotic cells. This theory posits that these organelles were once free-living prokaryotic organisms that were engulfed by a larger host cell. Over time, a symbiotic relationship developed, with the engulfed prokaryotes providing energy (mitochondria) or synthesizing food (chloroplasts) for the host cell, and the host cell providing protection and resources.
Evidence supporting this theory includes the observation that mitochondria and chloroplasts have their own DNA and ribosomes, which resemble those of prokaryotes. They also reproduce independently within the host cell. This theory provides a plausible explanation for the origin of some key eukaryotic organelles and highlights the role of symbiotic relationships in the evolution of complex cells.
Cellular Differentiation and Cell Theory
Cellular differentiation, the process by which a single cell gives rise to a diverse array of specialized cell types, is a fundamental aspect of multicellular life and a crucial component of our understanding of cell theory. This process, driven by intricate molecular mechanisms, challenges and refines the classical tenets of cell theory by demonstrating the remarkable plasticity and functional diversity inherent within a single genome.
The following sections explore the process of cellular differentiation, its underlying genetic and epigenetic regulation, and its implications for cell theory, disease, and therapeutic interventions.
Cellular Differentiation Process: A Detailed Description
Cellular differentiation begins with a totipotent zygote, a single cell capable of developing into an entire organism. Through a series of precisely orchestrated cell divisions and developmental stages, including the morula (a solid ball of cells), the blastocyst (a hollow ball of cells with an inner cell mass), and the gastrula (characterized by the formation of germ layers), the totipotent cell gives rise to progressively more specialized cell types.
Key molecular mechanisms driving this process include transcription factors, which bind to specific DNA sequences to regulate gene expression; epigenetic modifications, such as DNA methylation and histone modifications, which alter chromatin structure and gene accessibility; and intricate signaling pathways, which coordinate cell communication and differentiation decisions. For example, the hematopoietic stem cell lineage differentiates into various blood cells (erythrocytes, leukocytes, etc.), each with distinct functions, while neural stem cells give rise to the diverse cell types of the nervous system (neurons, glial cells, etc.).
Cellular Differentiation Process: A Visual Representation
Imagine a flowchart beginning with a single totipotent cell (zygote). The first branch point represents the decision to form the inner cell mass (embryonic stem cells) or trophoblast (placenta). Subsequent branch points depict decisions leading to the three primary germ layers (ectoderm, mesoderm, endoderm), each giving rise to distinct cell lineages. Each branch is influenced by signaling molecules (e.g., growth factors, morphogens) and intrinsic factors (e.g., transcription factor expression).
The flowchart visually depicts how environmental cues and internal programming interact to direct cellular fate, highlighting the non-linear and often probabilistic nature of differentiation.
Cellular Differentiation Process: A Comparative Analysis
Comparing the differentiation of a neuron and a hepatocyte reveals significant differences. Neurons, specialized for electrical signaling, express high levels of genes encoding ion channels, neurotransmitters, and structural proteins, resulting in their characteristic elongated morphology and synaptic connections. Hepatocytes, liver cells responsible for detoxification and metabolic processes, express high levels of genes encoding enzymes involved in these functions and possess a unique cellular architecture, including extensive endoplasmic reticulum and numerous mitochondria.
These distinct gene expression profiles and cellular structures reflect the specialized functions of these cell types, despite their shared origin from a single zygote.
Gene Regulation During Cellular Differentiation
Cellular differentiation profoundly impacts gene expression at all levels. Transcriptional regulation, mediated by transcription factors, determines which genes are transcribed into mRNA. Post-transcriptional mechanisms, such as mRNA splicing and stability, further modulate gene expression. Translational regulation controls the rate of protein synthesis from mRNA. For instance, the myoD transcription factor is crucial for muscle cell differentiation, activating the expression of muscle-specific genes.
Conversely, genes associated with other cell lineages are repressed.
Epigenetic Mechanisms in Cellular Differentiation
Epigenetic modifications play a critical role in regulating gene expression during differentiation. DNA methylation, the addition of a methyl group to DNA, and histone modifications, such as acetylation and methylation, can alter chromatin structure, making genes more or less accessible to transcriptional machinery. These modifications can be inherited, contributing to cellular memory and ensuring that differentiated cells maintain their specialized identity.
For example, DNA methylation patterns established during development can influence gene expression in differentiated cells, contributing to tissue-specific gene expression patterns.
Table of Gene Expression Levels Across Different Cell Types
| Gene | Cell Type | mRNA Level (relative) | Protein Level (relative) ||—————|————–|———————–|————————-|| MyoD | Myocyte | High | High || MyoD | Hepatocyte | Low | Low || MyoD | Neuron | Very Low | Very Low || Albumin | Hepatocyte | High | High || Albumin | Myocyte | Low | Low || Albumin | Neuron | Very Low | Very Low || Neurofilament | Neuron | High | High || Neurofilament | Hepatocyte | Low | Low || Neurofilament | Myocyte | Very Low | Very Low |
Implications of Cellular Differentiation for Cell Theory
Our understanding of cellular differentiation has significantly expanded and refined the classical cell theory. The classical view emphasized the fundamental unit of life as a single, self-contained cell. Cellular differentiation demonstrates that cells can exhibit remarkable diversity in structure and function while maintaining a common genetic origin. This highlights the dynamic nature of cells and the complex interplay between genes, environment, and cellular fate.
Disease Implications of Cellular Differentiation Errors
Errors in cellular differentiation can lead to various developmental disorders and diseases. For example, defects in hematopoietic stem cell differentiation can result in blood disorders like leukemia. Errors in neural differentiation can contribute to neurodevelopmental disorders such as autism spectrum disorder. Cancer can also arise from defects in cellular differentiation, where cells lose their specialized identity and acquire uncontrolled growth properties.
Therapeutic Potential of Manipulating Cellular Differentiation
Manipulating cellular differentiation holds immense therapeutic potential. Regenerative medicine aims to use stem cells to generate replacement tissues and organs, repairing damaged tissues. In cancer treatment, targeting pathways involved in cancer cell differentiation can help suppress tumor growth. Current research explores strategies to reprogram cells into desired cell types for treating various diseases.
Ethical Considerations of Manipulating Cellular Differentiation
Manipulating cellular differentiation raises ethical considerations, particularly concerning reproductive technologies and gene editing. Concerns include the potential for unintended consequences, the equitable access to these technologies, and the potential misuse of these powerful tools. Careful consideration of ethical implications is crucial in guiding research and applications in this field.
Cell Communication and Cell Theory
Cell communication, the intricate process by which cells interact and exchange information, is fundamental to the functioning of multicellular organisms and, in a broader sense, deeply intertwined with the tenets of cell theory. Understanding these communication mechanisms reveals how cells coordinate their activities to maintain homeostasis and execute complex biological processes, ultimately supporting the idea of cells as the basic units of life.
The failure of cell communication can lead to a wide range of diseases, highlighting its crucial role in health and disease.Cell communication relies on a variety of mechanisms, often involving signaling pathways that transmit information from one cell to another. These pathways are highly regulated and involve the coordinated action of numerous molecules, including proteins, lipids, and ions.
The specificity and efficiency of these pathways ensure that the right signal reaches the correct target cell and elicits the appropriate response. This precise control is critical for the proper functioning of tissues and organs.
Signal Transduction Pathways
Signal transduction pathways represent the molecular mechanisms by which extracellular signals are converted into intracellular responses. These pathways often involve a cascade of events, where a signal is relayed from one molecule to another, ultimately leading to a change in gene expression, cellular metabolism, or other cellular processes. For example, the binding of a growth factor to a receptor on the cell surface can trigger a cascade of intracellular signaling events, culminating in cell proliferation.
Another example is the activation of immune cells through cytokine signaling, where the binding of cytokines to their receptors on immune cells initiates a signaling cascade that leads to immune responses. These pathways are highly specific and regulated, ensuring the appropriate response to the signal. Disruptions in these pathways can lead to various diseases.
Cell Communication in Multicellular Organisms
Cell communication is essential for the coordination of cellular activities in multicellular organisms. In animals, for instance, the nervous and endocrine systems rely heavily on cell communication to regulate various physiological processes. Hormones, secreted by endocrine glands, travel through the bloodstream to reach target cells, triggering specific responses. Similarly, neurotransmitters, released by neurons at synapses, transmit signals to other neurons or effector cells, enabling rapid communication within the nervous system.
This intricate interplay of signaling molecules allows for the coordinated function of different tissues and organs, enabling the organism to maintain homeostasis and respond to environmental changes.
Cell Communication and Cell Theory Support
The various mechanisms of cell communication directly support the tenets of cell theory. The fact that cells communicate with each other highlights their interdependence and demonstrates that they function as integrated units within a larger organism. The complexity of cell communication underscores the highly organized nature of cells and their ability to carry out sophisticated functions. Moreover, the precision and specificity of signaling pathways reinforce the idea that cells are autonomous entities capable of responding to their environment and interacting with other cells in a coordinated manner.
The coordinated actions of cells, orchestrated through cell communication, form the basis of multicellular organization and demonstrate the validity of the cell theory’s principle of cells as the fundamental units of life.
Cell Death and Cell Theory
Cell death, a seemingly contradictory concept within the framework of cell theory focused on cell growth and division, is now understood as a crucial and regulated process essential for multicellular life. Far from being a passive event, cell death is actively controlled, playing a vital role in development, tissue homeostasis, and the overall health of an organism. This section will explore the two primary types of cell death – apoptosis and necrosis – and their implications for our understanding of cell theory.
Considering the foundational principles of cell theory, one might initially overlook the complexities of inter-familial dynamics. Understanding the core tenets, however, is crucial; for example, spontaneous generation isn’t a part of modern cell theory. This contrasts sharply with the intricate family systems described in Bowen’s theory, where understanding the role of “dividers” is key, as explained in detail here: what do dividers do in bowens theory of families.
Returning to cellular biology, the consistent application of cell theory helps us accurately assess the life cycle of cells and the origins of life itself.
Apoptosis: Morphological and Biochemical Characteristics
Apoptosis, or programmed cell death, is a tightly regulated process characterized by distinct morphological and biochemical changes. These changes differ significantly from those observed in necrosis, a form of cell death resulting from injury or trauma. The following table highlights key distinctions:
Characteristic | Apoptosis | Necrosis |
---|---|---|
Cell Size | Shrinkage | Swelling |
Plasma Membrane | Intact, blebbing | Disrupted, leaky |
Cellular Contents | Apoptotic bodies formed, orderly degradation | Released into surrounding tissue, causing inflammation |
DNA Fragmentation | Internucleosomal fragmentation (ladder pattern on gel electrophoresis) | Random fragmentation |
Inflammation | Absent or minimal | Significant |
Apoptosis: Intrinsic and Extrinsic Pathways
Apoptosis is initiated through two major pathways: the intrinsic (mitochondrial) pathway and the extrinsic (death receptor) pathway.The intrinsic pathway is triggered by intracellular stress, such as DNA damage or cellular dysfunction. Key molecules involved include: Bcl-2 family proteins (pro-apoptotic Bax/Bak and anti-apoptotic Bcl-2/Bcl-xL), cytochrome c, Apaf-1, and caspase-9. A flowchart would illustrate cytochrome c release from mitochondria, leading to apoptosome formation and caspase-9 activation.The extrinsic pathway is initiated by extracellular signals binding to death receptors (e.g., Fas, TNF receptor).
Key molecules include: death ligands (e.g., FasL, TNF), adaptor proteins (e.g., FADD), and caspase-8. A flowchart would show ligand binding, receptor trimerization, FADD recruitment, and caspase-8 activation.
Apoptosis: The Role of Caspases
Caspases are a family of cysteine-aspartic acid proteases that play a central role in executing the apoptotic program. Initiator caspases (e.g., caspase-8, caspase-9) activate effector caspases (e.g., caspase-3, caspase-7), which cleave various cellular substrates, leading to the characteristic morphological and biochemical changes of apoptosis.
Necrosis: Types and Mechanisms
Necrosis is characterized by uncontrolled cell death resulting from various injuries. Different types of necrosis exist, including coagulative (common in ischemic injury), liquefactive (characteristic of brain infarcts), and caseous (seen in tuberculosis). These types are distinguished by their morphological features and underlying causes, reflecting the nature of the cellular insult. For example, coagulative necrosis preserves the basic tissue architecture, while liquefactive necrosis results in complete tissue liquefaction.
[Detailed description of microscopic features of each necrosis type would be included here, including descriptions of cellular changes such as nuclear pyknosis, karyorrhexis, and karyolysis].ATP depletion, calcium influx, and membrane damage are key molecular mechanisms involved in necrosis. The loss of ATP compromises cellular function, leading to ion imbalance and ultimately, membrane rupture.
Necrosis: Inflammatory Response
Unlike apoptosis, necrosis triggers a strong inflammatory response. This is because the release of cellular contents from damaged cells activates the innate immune system, leading to recruitment of inflammatory cells and tissue repair processes. Apoptosis, on the other hand, avoids this inflammatory response due to the orderly degradation of cellular components and the absence of cellular leakage.
Cell Death Regulation and Tissue Homeostasis
Cell death is tightly regulated at both the cellular and molecular levels. Pro-apoptotic and anti-apoptotic proteins maintain a balance, ensuring that cell death occurs only when necessary. External signals, such as growth factors and cytokines, also influence cell death pathways. Regulated cell death is crucial for various physiological processes, including development (e.g., digit formation), immune response (e.g., elimination of self-reactive lymphocytes), and tissue turnover.
Consequences of Dysregulated Cell Death
Dysregulation of cell death pathways can have severe consequences. Defects in apoptosis can lead to the accumulation of damaged cells, contributing to cancer development. Conversely, excessive apoptosis can cause tissue damage and contribute to autoimmune disorders and neurodegenerative diseases.
Implications for Cell Theory: Programmed Cell Death
The discovery of programmed cell death has significantly expanded our understanding of cell theory. It challenges the traditional view of cell division as the sole mechanism controlling cell population size, demonstrating that active cell elimination is equally crucial.
Implications for Cell Theory: Cell Differentiation and Tissue Development
Apoptosis plays a critical role in shaping tissue architecture and function during development. For example, apoptosis is essential for the formation of the fingers and toes during embryogenesis. The removal of interdigital cells by apoptosis allows for the separation of digits.
Implications for Cell Theory: Cellular Senescence and Aging
Cell death is intricately linked to cellular senescence and organismal aging. The accumulation of senescent cells, which are unable to divide but resist apoptosis, contributes to tissue dysfunction and age-related diseases. The balance between cell proliferation, cell death, and cellular senescence is a crucial factor in determining lifespan.
Cell Theory and Cancer
Cancer fundamentally challenges the core tenets of cell theory, which posits that all living organisms are composed of cells, cells are the basic unit of life, and all cells arise from pre-existing cells. The uncontrolled proliferation and aberrant behavior of cancer cells directly contradict the regulated processes of cell division, differentiation, and death described by cell theory. Understanding this conflict is crucial for developing effective cancer treatments.
Cell Theory Violation in Cancer
The table below contrasts the behavior of normal cells with that of cancerous cells, highlighting the violations of cell theory principles.
Feature | Normal Cell Behavior | Cancerous Cell Behavior |
---|---|---|
Cell Division | Highly regulated; controlled by checkpoints (e.g., G1, G2, M) and signaling pathways ensuring accurate DNA replication and chromosome segregation. Cell division only occurs when appropriate signals are received and necessary conditions are met. | Uncontrolled and rapid; bypasses checkpoints and signaling pathways. Cells divide regardless of the presence of growth signals or the integrity of DNA. This leads to the formation of tumors. |
Cell Differentiation | Cells differentiate into specialized cell types with specific functions, contributing to tissue homeostasis and organismal function. This process is carefully orchestrated by genetic and epigenetic mechanisms. | Loss of differentiation (anaplasia); cells revert to an undifferentiated state, lacking specialized functions and exhibiting abnormal morphology. This contributes to the invasive and metastatic properties of cancer. |
Cell Death (Apoptosis) | Programmed cell death is a crucial mechanism for eliminating damaged or unwanted cells, maintaining tissue integrity and preventing tumor formation. Apoptosis is tightly regulated by signaling pathways. | Reduced or absent apoptosis; damaged or abnormal cells survive and proliferate, leading to the accumulation of cancerous cells and tumor growth. This resistance to apoptosis is a hallmark of cancer. |
Cell Communication | Cells communicate with each other through various signaling pathways, coordinating their growth, differentiation, and death. This communication is essential for maintaining tissue homeostasis. | Disrupted or altered cell communication; cancer cells often ignore or misinterpret signals from neighboring cells, contributing to uncontrolled growth and invasion of surrounding tissues. They may also produce signals that promote their own growth and survival. |
Genetic Mutations and Cell Theory
Oncogenes and tumor suppressor genes play critical roles in regulating cell growth and division. Mutations in these genes disrupt the normal cellular processes governed by cell theory, leading to uncontrolled cell growth and cancer development.The RAS gene family, for instance, encodes proteins involved in signal transduction pathways that regulate cell growth and division. Mutations in RAS genes can lead to constitutive activation of these pathways, resulting in uncontrolled cell proliferation.
Similarly, the p53 gene, a tumor suppressor gene, acts as a “guardian of the genome,” inducing cell cycle arrest or apoptosis in response to DNA damage. Mutations in p53 lead to the loss of its tumor-suppressing function, allowing cells with damaged DNA to proliferate and potentially become cancerous.A simplified diagram could illustrate this:[Description of Diagram: A diagram showing two pathways.
The normal pathway depicts a signal triggering a cascade involving RAS and p53, leading to either cell cycle arrest or apoptosis. The cancerous pathway shows a mutated RAS gene constantly activating the pathway, bypassing p53’s function and resulting in uncontrolled cell division.]
Cancer Cell Metabolism
Cancer cells exhibit altered metabolic processes compared to normal cells, a phenomenon known as the Warburg effect. This involves a shift from oxidative phosphorylation (aerobic respiration) to glycolysis, even in the presence of oxygen. This metabolic reprogramming provides cancer cells with the energy and building blocks needed for rapid proliferation, contributing to the violation of cell theory’s principles of regulated cell growth and division.
The increased reliance on glycolysis also supports the production of biosynthetic precursors required for rapid cell growth and division, further fueling uncontrolled proliferation.
Cancer Treatment Strategies and Cell Theory
Cancer treatments aim to restore or exploit the principles of cell theory to control cancer cell growth. Chemotherapy utilizes drugs that interfere with cell division, targeting rapidly dividing cancer cells. Radiation therapy damages DNA, inducing apoptosis in cancer cells. Targeted therapies aim to specifically inhibit the activity of oncogenes or restore the function of tumor suppressor genes, thus restoring the regulated processes governed by cell theory.
Cell Theory and Cancer Stem Cells
Cancer stem cells (CSCs) are a subpopulation of cancer cells with self-renewal and differentiation capabilities, contributing to tumor initiation, progression, and metastasis. These cells defy the principles of cell theory by exhibiting an extended lifespan and resistance to conventional therapies, challenging our understanding of regulated cell growth and death. Their unique properties, including enhanced DNA repair mechanisms and altered signaling pathways, contribute to treatment resistance and tumor recurrence.
Comparing Cancer Development Across Different Cell Types
Cancer development varies across different cell types due to differences in their genetic makeup, signaling pathways, and microenvironment.* Epithelial Cells: Epithelial cancers (carcinomas) are the most common type, often arising from mutations in genes regulating cell growth and differentiation. They frequently exhibit loss of cell polarity and tissue architecture.* Hematopoietic Cells: Hematopoietic cancers (leukemias and lymphomas) originate from blood-forming cells in the bone marrow.
These cancers often involve chromosomal translocations and mutations in genes regulating cell differentiation and survival. They typically display impaired cell differentiation and uncontrolled proliferation of immature blood cells.Both types demonstrate violations of cell theory principles, particularly regarding regulated cell division, differentiation, and apoptosis, but the specific genetic and epigenetic alterations differ, leading to distinct cancer phenotypes and treatment responses.
FAQ Summary
What are some common misconceptions about cell theory?
Common misconceptions include believing that all cells are identical, that all organisms have the same types of cells, or that viruses are considered living organisms under cell theory.
Does cell theory apply to all living organisms?
While cell theory is a fundamental principle, certain acellular entities like viruses challenge its strict application, prompting ongoing discussion about the definition of life itself.
How does the creation of synthetic cells impact cell theory?
Synthetic cells raise questions about the origin of life and the definition of a “living” cell, potentially requiring further refinement of cell theory.