Which discovery supported the endosymbiotic theory? The answer lies not in a single eureka moment, but in the convergence of multiple lines of evidence revealing striking similarities between mitochondria and chloroplasts and free-living prokaryotes. This theory, proposing that these organelles originated from symbiotic bacteria engulfed by ancestral eukaryotic cells, is supported by a wealth of data spanning structural analysis, genetic comparisons, metabolic pathways, and antibiotic sensitivities.
Examining these converging lines of evidence provides a compelling narrative of cellular evolution.
The double membrane structure of mitochondria and chloroplasts, mirroring the inner and outer membranes of bacteria, offers a crucial initial clue. Further support emerges from the presence of their own distinct DNA (mtDNA and cpDNA), which shares similarities with bacterial genomes and encodes essential organelle proteins. The ribosomes within these organelles, resembling bacterial 70S ribosomes in size and sensitivity to antibiotics, further strengthen the endosymbiotic hypothesis.
Comparative genomic analyses reveal significant sequence homology between organellar and bacterial genomes, providing robust phylogenetic evidence. The independent division of mitochondria and chloroplasts via binary fission, akin to bacterial cell division, further supports their independent origins. Finally, the observation that these organelles exhibit sensitivity to certain antibiotics that target bacterial ribosomes solidifies the connection to prokaryotic ancestors.
Mitochondrial Structure and Function
The intricate structure and function of mitochondria provide compelling evidence for the endosymbiotic theory, a cornerstone of evolutionary biology. This theory posits that mitochondria originated from free-living bacteria that were engulfed by an ancestral eukaryotic cell, forming a mutually beneficial symbiotic relationship. The following sections will detail the key structural and functional features of mitochondria that strongly support this revolutionary concept.
Double Membrane Structure and Endosymbiotic Theory
Mitochondria possess a distinctive double membrane structure. The outer membrane is relatively permeable, while the inner membrane is highly folded into cristae, creating a large surface area. The space between these membranes is called the intermembrane space, and the innermost compartment is the matrix. This double membrane is a critical piece of evidence for the endosymbiotic theory. The outer membrane is thought to have arisen from the engulfing process of the ancestral eukaryotic cell, while the inner membrane represents the original bacterial plasma membrane of the engulfed prokaryote.
Mitochondrial DNA (mtDNA) and the Endosymbiotic Hypothesis
Mitochondria contain their own circular DNA molecule, mtDNA, separate from the nuclear DNA. mtDNA is relatively small and encodes a limited number of genes primarily involved in mitochondrial protein synthesis and oxidative phosphorylation. The presence of a distinct genome within the mitochondrion is strong evidence for its endosymbiotic origin, as it reflects the genetic material of the original bacterial ancestor.
The genetic code of mtDNA differs slightly from the universal genetic code used by nuclear DNA, further supporting its independent evolutionary history.
Feature | mtDNA | Nuclear DNA |
---|---|---|
Shape | Circular | Linear |
Size | Small (16.5 kb in humans) | Large (3 billion bp in humans) |
Genetic Code | Slightly different from standard code | Standard genetic code |
Gene Content | Primarily genes for oxidative phosphorylation | Vast array of genes |
Mitochondrial vs. Cytoplasmic Ribosomes
Mitochondria possess their own ribosomes, mitoribosomes, which are distinct from cytoplasmic ribosomes. Mitoribosomes are smaller (70S) than cytoplasmic ribosomes (80S) and more closely resemble bacterial ribosomes in size and sensitivity to certain antibiotics. The rRNA composition of mitoribosomes also shows similarities to bacterial rRNA, providing further support for the endosymbiotic theory.
Feature | Mitoribosomes | Cytoplasmic Ribosomes |
---|---|---|
Size | 70S | 80S |
Sedimentation Coefficient | 70S | 80S |
Antibiotic Sensitivity | Similar to bacterial ribosomes | Different from bacterial ribosomes |
rRNA Composition | Similar to bacterial rRNA | Different from bacterial rRNA |
Mitochondrial Protein Synthesis and Endosymbiotic Implications
Mitochondrial protein synthesis involves mtDNA, mitoribosomes, mitochondrial tRNA, and mitochondrial mRNA. However, a significant portion of mitochondrial proteins are encoded by nuclear DNA, synthesized in the cytoplasm, and then imported into the mitochondria. This complex interplay between nuclear and mitochondrial genomes reflects the evolutionary integration of the endosymbiont into the eukaryotic cell.
Cristae Structure and Function
Mitochondrial cristae exhibit diverse morphologies, including lamellar, tubular, and vesicular structures. These variations are correlated with the metabolic activity of the mitochondria. The extensive surface area provided by cristae is crucial for the efficient functioning of the electron transport chain and ATP synthesis. Cristae structure directly influences the efficiency of energy production.
Mitochondrial Dynamics
Mitochondria undergo continuous cycles of fission (division) and fusion (merging), processes regulated by specific proteins. These dynamic processes are essential for mitochondrial quality control, ensuring the removal of damaged mitochondria and the distribution of healthy mitochondria to meet cellular energy demands. Impaired mitochondrial dynamics contribute to various pathologies.
Mitochondrial Dysfunction and Disease
Mitochondrial dysfunction is implicated in a wide range of human diseases, including mitochondrial myopathies, Leigh syndrome, and MELAS syndrome. These diseases result from mutations in mtDNA or nuclear genes encoding mitochondrial proteins, leading to impaired oxidative phosphorylation and energy production.
Disease | Molecular Mechanism | Pathological Consequences |
---|---|---|
Mitochondrial Myopathies | Mutations in mtDNA or nuclear genes affecting oxidative phosphorylation | Muscle weakness, fatigue |
Leigh Syndrome | Mutations in genes encoding mitochondrial enzymes | Neurological dysfunction, lactic acidosis |
MELAS Syndrome | Mutation in mtDNA affecting tRNA | Mitochondrial encephalomyopathy, lactic acidosis, stroke-like episodes |
Chloroplast Structure and Function
The chloroplast, the powerhouse of plant cells, stands as a crucial piece of evidence supporting the endosymbiotic theory. Its complex internal structure and unique genetic makeup mirror those of free-living bacteria, offering a compelling narrative of evolutionary history. A closer examination reveals a fascinating tale of cellular cooperation and evolutionary adaptation, a tale that challenges simplistic explanations of cellular origins.Chloroplast Internal Structure and FunctionThe chloroplast’s internal architecture is remarkably intricate, reflecting its multifaceted role in photosynthesis.
Encased within a double membrane, the chloroplast contains a system of interconnected membranous sacs known as thylakoids. These thylakoids are stacked into grana, increasing the surface area available for light-harvesting complexes. The space surrounding the thylakoids is called the stroma, a fluid-filled region containing enzymes, ribosomes, and chloroplast DNA (cpDNA). This compartmentalization is not accidental; it is essential for the efficient execution of photosynthesis.
The thylakoid membranes house the photosynthetic electron transport chain, the site of light-dependent reactions, while the stroma is the location of the carbon fixation reactions (Calvin cycle). This intricate division of labor ensures optimal energy conversion and carbohydrate synthesis.
Chloroplast DNA and the Endosymbiotic Theory
The presence of cpDNA within chloroplasts provides powerful support for the endosymbiotic theory. cpDNA is a circular molecule, similar to the genomes of bacteria, and encodes for essential proteins involved in photosynthesis and chloroplast function. This independent genome strongly suggests that chloroplasts originated from an ancient endosymbiotic event, where a photosynthetic cyanobacterium was engulfed by a eukaryotic cell. The retention of a distinct genome within the chloroplast, despite billions of years of co-evolution, is a testament to the enduring legacy of this symbiotic partnership.
The genetic analysis of cpDNA, comparing it to extant cyanobacteria, continues to refine our understanding of this pivotal evolutionary event. The significant similarities are difficult to explain through any other evolutionary mechanism, making the endosymbiotic theory the most parsimonious explanation.
Chloroplast Ribosomes and Their Cellular Context
Chloroplasts contain 70S ribosomes, a size characteristic of bacterial ribosomes, distinctly different from the 80S ribosomes found in the eukaryotic cytoplasm. Mitochondria, another endosymbiotic organelle, also possess 70S ribosomes. This ribosomal similarity between chloroplasts, mitochondria, and bacteria further reinforces the endosymbiotic hypothesis. The difference in ribosome size reflects a fundamental distinction in protein synthesis machinery, indicating the independent evolutionary history of these organelles.
This independent protein synthesis capacity within chloroplasts adds another layer to their semi-autonomous nature. The fact that chloroplasts retain the capacity for independent protein synthesis is a critical observation that strengthens the argument for their independent origins.
Photosynthesis and the Independent Origin of Chloroplasts
Photosynthesis, the process by which light energy is converted into chemical energy in the form of glucose, is a defining characteristic of chloroplasts. The light-dependent reactions occur within the thylakoid membranes, involving chlorophyll and other pigment molecules that capture light energy. This energy is used to generate ATP and NADPH, which are then utilized in the light-independent reactions (Calvin cycle) in the stroma to fix carbon dioxide into organic molecules.
The remarkable efficiency and complexity of photosynthesis, coupled with the unique structural and genetic features of chloroplasts, strongly support their independent origin as endosymbionts. The evolutionary acquisition of photosynthesis through endosymbiosis represents a transformative event in the history of life on Earth, paving the way for the evolution of complex plant life. The highly specific and optimized nature of the photosynthetic apparatus further supports the idea that this system evolved within a self-contained entity, rather than arising de novo within a eukaryotic cell.
Genetic Similarities
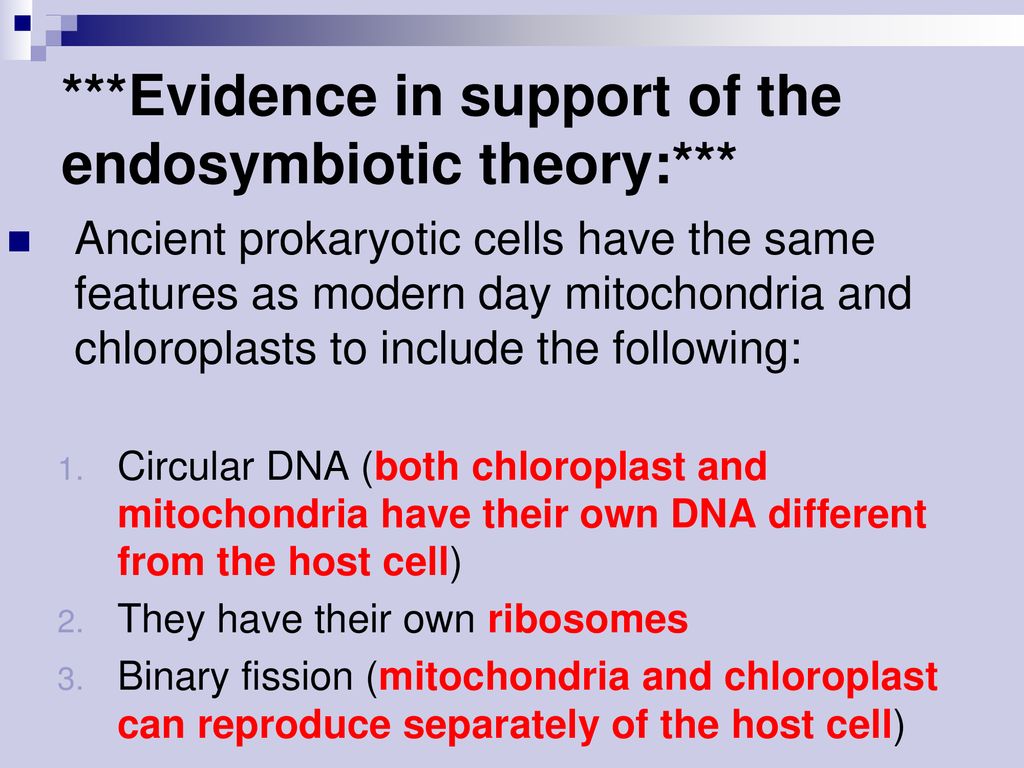
The endosymbiotic theory, while elegantly explaining the origin of mitochondria and chloroplasts, has faced its share of skepticism. The clinching evidence, however, lies not just in structural similarities but in the undeniable genetic fingerprints these organelles share with their free-living prokaryotic ancestors. Examining these genetic parallels provides a powerful, albeit often politically contested, argument for the theory’s validity.
The sheer volume of evidence makes ignoring these genetic echoes impossible for any serious scientist.Mitochondria and chloroplasts possess their own distinct genomes, separate from the nuclear DNA of their eukaryotic hosts. This genetic autonomy, a relic of their independent existence, is a cornerstone of the endosymbiotic theory. The similarities between these organelle genomes and those of specific bacterial lineages provide compelling support for the theory.
The implications are far-reaching, challenging long-held assumptions about the evolution of eukaryotic cells and the very nature of life itself.
Mitochondrial and Alpha-Proteobacterial Genome Similarities
Mitochondrial genomes, though significantly reduced compared to their alpha-proteobacterial ancestors, retain striking similarities. These similarities extend beyond mere gene presence; they include conserved gene order, codon usage bias, and even the presence of introns in some mitochondrial genomes, reflecting their bacterial heritage. For example, the genes encoding ribosomal RNAs (rRNAs) and ribosomal proteins in mitochondria share high sequence homology with their counterparts in alpha-proteobacteria.
This homology extends to other essential genes involved in energy metabolism, such as those encoding components of the electron transport chain. Furthermore, the presence of specific genetic markers, like particular tRNA genes, strengthens the phylogenetic link. The argument that these similarities are mere coincidences is weak and unsupported by the sheer weight of the evidence.
Chloroplast and Cyanobacterial Genome Similarities
The genetic relationship between chloroplasts and cyanobacteria is similarly compelling. Chloroplast genomes, like mitochondrial genomes, exhibit remarkable conservation with their cyanobacterial counterparts. The genes involved in photosynthesis, including those encoding photosystems I and II, as well as the ATP synthase, show strong sequence similarity and functional conservation. The presence of specific operons, characteristic of cyanobacteria, further underscores the evolutionary connection.
The size and structure of chloroplast genomes, while reduced from their ancestral cyanobacterial forms, still reflect their prokaryotic origins. This evidence, far from being circumstantial, is overwhelming and leaves little room for alternative explanations.
Comparison of Genetic Codes
A critical point of comparison lies in the genetic code itself. While the standard genetic code is largely universal, deviations exist. Mitochondrial and chloroplast genetic codes often differ from the standard code used in the host cell’s nucleus, but interestingly, often resemble the genetic codes found in their respective prokaryotic relatives. These subtle but significant variations in codon usage further solidify the endosymbiotic hypothesis.
This divergence is not arbitrary; it reflects the independent evolutionary trajectories of these organelles and their bacterial ancestors. Attempts to dismiss this evidence as mere random mutation are statistically improbable and intellectually dishonest.
Summary of Genetic Comparisons
Organelle | Genome Similarity | Evolutionary Lineage | Supporting Evidence |
---|---|---|---|
Mitochondria | High sequence similarity with alpha-proteobacteria in genes for respiration and ribosome components. | Derived from an alpha-proteobacterium. | Sequence homology of rRNA, tRNA, and protein-coding genes; conserved gene order; similar codon usage bias. |
Chloroplasts | High sequence similarity with cyanobacteria in genes for photosynthesis and ribosome components. | Derived from a cyanobacterium. | Sequence homology of photosynthetic genes; presence of cyanobacterial-like operons; similar codon usage bias. |
Ribosomal Structure and Function
The structure and function of ribosomes within mitochondria and chloroplasts provide compelling evidence supporting the endosymbiotic theory, which posits that these organelles originated from free-living bacteria. The remarkable similarities between organellar and bacterial ribosomes, while accompanied by key differences reflecting adaptation to the eukaryotic environment, paint a powerful picture of evolutionary history.
Mitochondrial Ribosomes
Mitochondrial ribosomes, or mitoribosomes, are responsible for synthesizing a subset of mitochondrial proteins crucial for oxidative phosphorylation and other mitochondrial functions. Mitoribosomes are smaller than their cytoplasmic counterparts, exhibiting a structure analogous to bacterial 70S ribosomes but with distinct compositional differences. The mitoribosome consists of a small subunit (mS) and a large subunit (mL). The mS subunit typically contains a single 12S rRNA molecule and numerous proteins, while the mL subunit possesses a 16S rRNA molecule and a larger complement of proteins.
The precise number of proteins varies across species. Mitoribosomes translate mRNAs encoding proteins essential for the electron transport chain, ATP synthase, and other components of the oxidative phosphorylation machinery. The unique challenges of mitochondrial protein synthesis include the import of many proteins from the cytoplasm and the reliance on a specialized protein import machinery. The confined environment of the mitochondrion, with its limited space and specialized metabolism, further complicates protein synthesis.A textual representation of a mitoribosome would show a small subunit (mS) depicted as a smaller oval shape, containing a 12S rRNA molecule represented as a coiled strand within the oval.
The larger subunit (mL), depicted as a larger oval, would contain a 16S rRNA molecule similarly represented, along with numerous protein molecules depicted as smaller circles scattered within the larger oval. The two subunits are shown closely associated, with the 16S rRNA of the mL subunit appearing to partially overlap with the 12S rRNA of the mS subunit.
Chloroplast Ribosomes
Chloroplast ribosomes, or chlororibosomes, share structural and functional similarities with mitoribosomes and bacterial ribosomes, reflecting their shared evolutionary ancestry. These ribosomes are responsible for synthesizing a significant portion of the proteins needed for photosynthesis and other chloroplast functions. The chlororibosome, similar to the mitoribosome, comprises a small subunit (cS) and a large subunit (cL). The cS subunit generally contains a 16S rRNA molecule and various proteins, while the cL subunit includes a 23S rRNA molecule and a larger protein complement.
Chloroplast protein synthesis involves specific targeting signals that direct newly synthesized proteins to their correct locations within the chloroplast. This process is critical for maintaining the structural and functional integrity of the chloroplast.A textual representation of a chlororibosome would mirror the mitoribosome description, but with the cS subunit containing a 16S rRNA and the cL subunit containing a 23S rRNA.
The overall size and shape would be similar to the mitoribosome depiction, but potentially slightly larger to reflect the slightly larger size of chlororibosomes compared to mitoribosomes.
Comparative Analysis
Mitochondrial and chloroplast ribosomes, while sharing ancestry with bacterial ribosomes, exhibit size and compositional differences. Bacterial 70S ribosomes are composed of a 30S and a 50S subunit. The sedimentation coefficients (Svedberg units) reflect these size differences. Bacterial 70S ribosomes are approximately 70S, with the 30S subunit and the 50S subunit having their own distinct sizes. Mitochondrial ribosomes are typically smaller than bacterial ribosomes, ranging from 55S to 70S depending on the species, while chloroplast ribosomes are generally similar in size to bacterial ribosomes.
The precise rRNA and protein composition also varies among species.
Organism | Subunit Size | Number of rRNA Molecules per Subunit | Total Number of Proteins per Ribosome |
---|---|---|---|
*E. coli* | 30S, 50S | 1 (16S), 2 (23S, 5S) | ~55 |
Human Mitochondria | mS, mL | 1 (12S), 1 (16S) | ~78 |
*Arabidopsis* Chloroplast | cS, cL | 1 (16S), 2 (23S, 5S) | ~70 |
The 16S rRNA (or its equivalent) sequence shows significant similarity between bacterial and organellar ribosomes, providing strong support for the endosymbiotic hypothesis. However, some variations exist reflecting evolutionary divergence and adaptation to the eukaryotic cellular environment.
Endosymbiotic Theory Implications
The striking similarities in ribosomal structure and composition between bacteria and mitochondria/chloroplasts strongly support the endosymbiotic theory. The presence of 70S-like ribosomes in these organelles, closely resembling those of bacteria, provides powerful evidence for their bacterial ancestry. The differences observed reflect adaptations to the eukaryotic environment, including changes in protein composition and gene expression regulation.Counterarguments to the endosymbiotic theory based on ribosomal characteristics are few and largely address the nuances of evolutionary adaptation and the complexities of ribosomal evolution.
The observed differences are more easily explained by evolutionary divergence following endosymbiosis than by alternative hypotheses.
Ribosomal RNA Sequence Comparison
Organism | rRNA Gene | Sequence Similarity (%) toE. coli* 16S rRNA | Sequence Similarity (%) to Human Mitochondrial 12S rRNA | Sequence Similarity (%) to
|
---|---|---|---|---|
*E. coli* | 16S rRNA | 100 | ~50 | ~70 |
Human Mitochondria | 12S rRNA | ~50 | 100 | ~40 |
*Arabidopsis thaliana* Chloroplast | 16S rRNA | ~70 | ~40 | 100 |
Note: These percentages are approximate and vary depending on the specific strains and analysis methods used. The actual values can be found in published phylogenetic studies.
Division and Reproduction
The independent replication and division of mitochondria and chloroplasts represent a cornerstone of the endosymbiotic theory, a theory that continues to spark debate and refine our understanding of eukaryotic cell evolution. The mechanisms underlying organelle division, strikingly similar to bacterial binary fission, offer compelling evidence for their prokaryotic origins. This section will dissect the processes involved, highlighting both similarities and crucial differences, and ultimately strengthening the case for endosymbiosis.
Binary Fission in Mitochondria and Chloroplasts
Mitochondria and chloroplasts, the powerhouses and solar panels of eukaryotic cells respectively, reproduce through a process remarkably similar to bacterial binary fission. This autonomous replication, independent of the host cell’s nuclear cycle, is a critical piece of evidence supporting the endosymbiotic theory. The process involves DNA replication, segregation of the replicated genomes, and finally, cytokinesis – the division of the organelle into two daughter organelles.
Binary Fission in Mitochondria
Mitochondrial binary fission is a complex process involving multiple steps and proteins. DNA replication, initiated at multiple origins, is followed by segregation of the replicated genomes, often facilitated by proteins analogous to bacterial partitioning systems. These systems ensure each daughter mitochondrion receives a complete genome. Finally, cytokinesis involves membrane invagination and fission, resulting in the separation of the two daughter mitochondria.
While specific proteins involved are still under investigation, homologs of bacterial proteins like MinCDE and FtsZ have been implicated in the process. The precise choreography of these proteins, however, remains an area of active research, with many details still needing clarification.
Binary Fission in Chloroplasts
Chloroplast binary fission shares many similarities with mitochondrial fission, yet also exhibits distinct characteristics. Like mitochondria, chloroplasts replicate their DNA and segregate the genomes before cytokinesis. However, the specific proteins involved and the timing of events can differ. The process is further complicated by the presence of the thylakoid membrane system, an intricate internal membrane network crucial for photosynthesis.
The division of this system requires precise coordination during chloroplast cytokinesis, a process not fully understood. While homologs of bacterial division proteins have been identified in chloroplasts, the extent of their functional conservation and the precise mechanisms of thylakoid division remain topics of ongoing research and debate.
Comparison of Mitochondrial and Chloroplast Binary Fission
Step | Mitochondrial Binary Fission | Chloroplast Binary Fission |
---|---|---|
DNA Replication | Multiple origins, likely involving bacterial-like replication machinery. | Multiple origins, likely involving bacterial-like replication machinery, potentially with some eukaryotic modifications. |
Segregation | Involves proteins analogous to bacterial partitioning systems; precise mechanisms are still being investigated. | Involves proteins with homology to bacterial partitioning systems, but the details are less well understood than in mitochondria. |
Cytokinesis | Membrane invagination and fission, involving proteins analogous to bacterial FtsZ. | Membrane invagination and fission, involving proteins analogous to bacterial FtsZ, with additional complexities due to thylakoid membrane division. |
Comparison with Bacterial Cell Division
The striking resemblance between organelle and bacterial binary fission strongly supports the endosymbiotic theory. The core mechanisms—DNA replication, segregation, and cytokinesis—are remarkably conserved.
Comparison of Binary Fission Mechanisms
Feature | *E. coli* Binary Fission | Mitochondrial Binary Fission | Chloroplast Binary Fission |
---|---|---|---|
DNA Replication | Single origin, highly regulated. | Multiple origins, less tightly regulated. | Multiple origins, less tightly regulated. |
Segregation | Well-characterized partitioning systems (e.g., ParA/ParB). | Homologs of bacterial partitioning proteins identified, but details are incomplete. | Homologs of bacterial partitioning proteins identified, but details are incomplete. |
Cytokinesis | Involves FtsZ ring and other essential proteins. | Involves FtsZ homologs and other proteins; precise mechanisms still under investigation. | Involves FtsZ homologs and other proteins; precise mechanisms still under investigation, including thylakoid membrane division. |
Variations in Bacterial Binary Fission
Bacterial binary fission, while fundamentally conserved, exhibits variations across species. These variations involve the specific proteins involved in DNA replication, segregation, and cytokinesis. The diversity observed in bacteria mirrors the incomplete understanding of the specific proteins and mechanisms involved in organelle division. This complexity highlights the ongoing need for further research to fully elucidate the nuances of organelle division.
The remarkable similarities between the binary fission of mitochondria and chloroplasts and that of bacteria provide powerful evidence for the endosymbiotic theory. The conserved mechanisms suggest a common ancestry, reinforcing the hypothesis that these organelles evolved from free-living prokaryotes.
Support for the Endosymbiotic Theory
The independent division of mitochondria and chloroplasts, decoupled from the host cell cycle, provides strong evidence for their endosymbiotic origin. This autonomy suggests they retain remnants of their ancestral prokaryotic regulatory systems.
Genes Supporting Independent Replication and Division
Specific genes within the mitochondrial and chloroplast genomes encode proteins essential for their replication and division. These genes, often homologous to bacterial genes, further support the endosymbiotic theory. The presence of these genes, distinct from the host cell’s nuclear genome, reinforces the notion of independent evolutionary trajectories.
Exceptions and Challenges to the Simple Model
While the basic model of independent organelle division is compelling, exceptions and complexities exist. The degree of interaction between organelle and host cell division machinery, for instance, is a subject of ongoing investigation. These complexities do not invalidate the endosymbiotic theory but rather highlight the intricate evolutionary interplay between the endosymbiont and its host.
Illustration of Binary Fission
(Note: Detailed diagrams cannot be created in this text-based format. However, descriptions are provided below.)
Mitochondrial Binary Fission Diagram
The diagram would depict a mitochondrion undergoing binary fission. The initial stage would show a mitochondrion with its circular DNA molecule. The next stage would illustrate DNA replication, with two identical DNA molecules appearing. Then, the diagram would show the segregation of the replicated DNA, with each copy moving towards opposite poles of the organelle. The final stage would illustrate cytokinesis, where the mitochondrial membrane invaginates and eventually pinches off, forming two daughter mitochondria, each with a complete copy of the mitochondrial DNA.
The diagram would clearly label the mitochondrial DNA, the inner and outer mitochondrial membranes, and the sites of membrane invagination.
The discovery of mitochondria and chloroplasts possessing their own DNA strongly supported the endosymbiotic theory. Understanding how this evidence fits into the broader scientific framework requires considering what constitutes a robust theory, a process explained in detail by learning about what is theory and construction. This understanding then reinforces the significance of the independent genomes found within these organelles as compelling support for the endosymbiotic theory’s explanation of eukaryotic cell evolution.
Caption:
This diagram illustrates mitochondrial binary fission, highlighting the key steps: DNA replication, segregation, and cytokinesis. The process closely resembles bacterial binary fission, providing strong support for the endosymbiotic theory.
Chloroplast Binary Fission Diagram
The diagram would depict a chloroplast undergoing binary fission. Similar to the mitochondrial diagram, it would illustrate DNA replication, segregation, and cytokinesis. However, a key difference would be the inclusion of the thylakoid membrane system within the chloroplast. The diagram would show how this internal membrane system is also divided during cytokinesis, a more complex process than in mitochondria.
The diagram would clearly label the chloroplast DNA, the inner and outer chloroplast membranes, the thylakoid membranes, and the sites of membrane invagination.
Caption:
This diagram illustrates chloroplast binary fission, emphasizing the similarities and differences compared to mitochondrial binary fission. The division of the thylakoid membrane system adds complexity to the process, yet the overall mechanism remains consistent with bacterial binary fission.
Membrane Composition
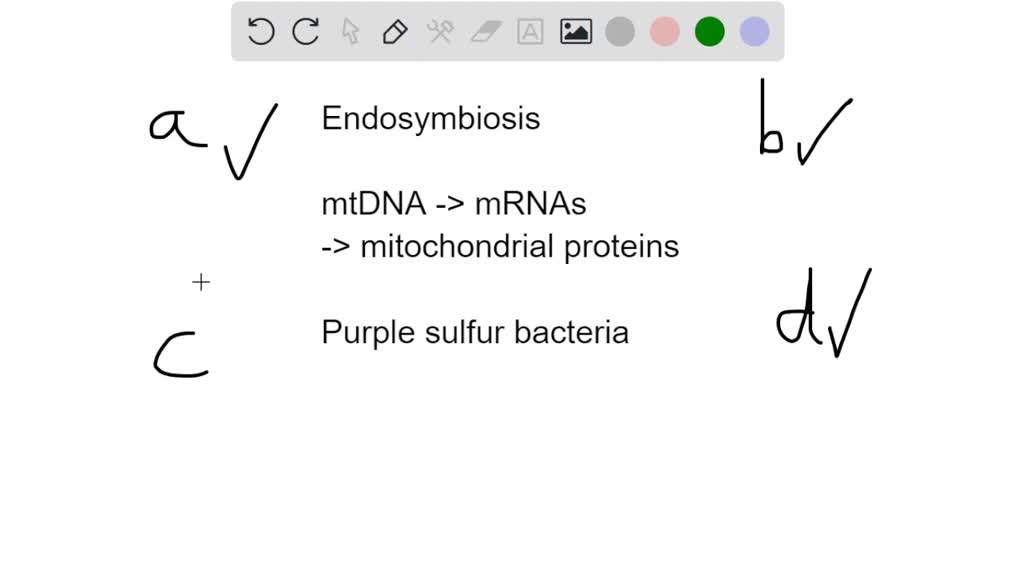
The lipid composition of mitochondrial and chloroplast membranes offers compelling evidence supporting the endosymbiotic theory, a cornerstone of evolutionary biology that posits these organelles originated from free-living bacteria. Examining these membranes reveals striking parallels with bacterial membranes, challenging the notion of a purely linear evolutionary pathway and highlighting the dynamic interplay of symbiotic relationships in shaping cellular architecture. This analysis will delve into the specifics of membrane lipid composition, exploring both similarities and differences, and their evolutionary significance.Mitochondrial and chloroplast membranes exhibit a lipid composition remarkably similar to that of bacterial membranes, particularly in their high proportion of phosphatidylethanolamine and cardiolipin.
These lipids are crucial for membrane fluidity and stability, and their presence in all three types of membranes strongly suggests a common evolutionary origin. Conversely, the relative abundance of other lipids, such as phosphatidylcholine, differs significantly between eukaryotic and bacterial membranes. These variations may reflect adaptations to the unique environments within the eukaryotic cell, highlighting the evolutionary pressures shaping membrane composition post-endosymbiosis.
Phospholipid Composition and its Evolutionary Implications
The inner mitochondrial membrane, for example, is characterized by a high proportion of cardiolipin, a dimeric phospholipid essential for maintaining the structural integrity and function of the electron transport chain. This lipid is also found in significant quantities in bacterial membranes, further reinforcing the link between mitochondria and their prokaryotic ancestors. Similarly, chloroplast membranes share this characteristic with cyanobacteria, their presumed ancestors.
Differences in the relative abundance of other phospholipids, such as phosphatidylcholine, which is more abundant in eukaryotic membranes than in bacterial membranes, might reflect adaptations to the specific environmental conditions within the eukaryotic host cell, suggesting evolutionary divergence after the endosymbiotic event. The evolutionary pressures driving these changes could include factors like membrane fluidity requirements and interactions with other cellular components.
For instance, the increased abundance of phosphatidylcholine in eukaryotic membranes may enhance their interaction with other cellular membranes or proteins.
Cardiolipin’s Role in Membrane Function
Cardiolipin, a unique phospholipid, plays a crucial role in maintaining the structural integrity and functionality of the inner mitochondrial membrane. Its distinctive dimeric structure allows for tight packing of the membrane, contributing to the formation of cristae, the characteristic infoldings that increase the surface area for oxidative phosphorylation. The presence of cardiolipin in bacterial membranes supports the endosymbiotic theory, implying that this crucial lipid was already present in the ancestral prokaryote.
The conservation of this lipid across such diverse lineages is a powerful testament to its functional importance.
Diagram of Key Membrane Components
Imagine a diagram showing three cross-sections: a bacterial membrane, a mitochondrial inner membrane, and a chloroplast thylakoid membrane. Each membrane is depicted as a phospholipid bilayer, with the hydrophilic heads facing outwards and the hydrophobic tails inwards. The diagrams would highlight the presence of cardiolipin and phosphatidylethanolamine in all three membranes, emphasizing their shared ancestry. The differences in the relative abundance of other phospholipids, like phosphatidylcholine, would also be visually represented, illustrating the evolutionary divergence that occurred after the endosymbiotic event.
The presence of specific membrane proteins unique to each membrane type would further emphasize the evolutionary adaptations that have occurred in each lineage. This visual representation would clearly demonstrate the similarities and differences in membrane composition, providing a powerful illustration of the evidence supporting the endosymbiotic theory.
Metabolic Pathways
The striking similarities between the metabolic pathways of mitochondria and chloroplasts and those of free-living bacteria provide compelling evidence for the endosymbiotic theory. These shared pathways are not merely coincidental; they represent a direct inheritance from the ancestral prokaryotes that were engulfed billions of years ago. The persistence of these ancient metabolic processes within these organelles is a testament to their functional importance and evolutionary conservation.
Examining these pathways reveals a powerful narrative of cellular evolution, a story written in the very chemistry of life itself.The presence of nearly identical metabolic pathways in mitochondria, chloroplasts, and bacteria strongly supports the hypothesis that these organelles evolved from free-living prokaryotes. These pathways are not just superficially similar; they often involve the same enzymes, intermediates, and regulatory mechanisms, indicating a common evolutionary origin.
The efficiency of these pathways in energy production and metabolic processing reinforces their conservation throughout evolutionary history. Any significant deviation would likely have been selected against due to the profound impact on cellular function.
Key Metabolic Pathways Shared Between Organelles and Bacteria
The following list details several key metabolic pathways found in both mitochondria, chloroplasts, and their bacterial ancestors. The near-identical nature of these pathways across vastly different organisms is a powerful argument for their shared evolutionary heritage, challenging any alternative explanations. The persistence of these ancient pathways provides a powerful molecular fossil record of the endosymbiotic event.
- Glycolysis: This fundamental pathway for glucose breakdown occurs in the cytoplasm of both eukaryotic cells and in bacteria. In mitochondria, glycolysis’s products feed into the citric acid cycle. The near-identical enzymatic machinery and metabolic intermediates in both systems offer compelling evidence of their shared ancestry. Any divergence would imply a significant and unlikely evolutionary leap.
- Citric Acid Cycle (Krebs Cycle): This central metabolic pathway, primarily located in the mitochondrial matrix, is virtually identical to the pathways found in many aerobic bacteria. The cycle’s role in generating reducing equivalents (NADH and FADH2) for oxidative phosphorylation is conserved across these lineages. The similarity is not limited to the overall process; the specific enzymes and their regulatory mechanisms show remarkable congruence.
- Oxidative Phosphorylation: The electron transport chain and ATP synthase, responsible for the majority of ATP production in eukaryotes, are remarkably similar in structure and function to those found in the bacterial plasma membrane. The proton gradient across the membrane, a key element in ATP synthesis, is a conserved feature across these systems. The high degree of similarity challenges explanations relying on convergent evolution.
- Photosynthesis (Chloroplasts): The light-dependent and light-independent reactions of photosynthesis in chloroplasts bear a striking resemblance to the photosynthetic pathways in cyanobacteria. This includes the use of similar pigments, electron transport chains, and carbon fixation mechanisms. The evolutionary link between chloroplasts and cyanobacteria is particularly compelling given the strong morphological and genetic similarities.
Evolutionary Connections: A Flow Chart of Metabolic Pathways
The following flow chart depicts the evolutionary connections between metabolic pathways in mitochondria, chloroplasts, and their bacterial ancestors. The chart illustrates the conservation of key pathways and their integration into the eukaryotic cell. The shared ancestry is visually represented by the branching pattern, emphasizing the evolutionary continuity.
A simplified representation:[Bacterial Glycolysis] –> [Mitochondrial Glycolysis][Bacterial Citric Acid Cycle] –> [Mitochondrial Citric Acid Cycle][Bacterial Electron Transport Chain] –> [Mitochondrial Electron Transport Chain][Cyanobacterial Photosynthesis] –> [Chloroplast Photosynthesis]
The remarkable conservation of these pathways across vastly different organisms is not easily dismissed as mere coincidence. It points towards a deep evolutionary relationship, providing strong support for the endosymbiotic theory. Alternative hypotheses fail to adequately explain this level of functional and structural similarity.
Antibiotic Sensitivity
The sensitivity of mitochondria and chloroplasts to certain antibiotics provides compelling evidence supporting the endosymbiotic theory, which posits that these organelles originated from free-living bacteria. This sensitivity stems from the retention of bacterial-like features within these organelles, including their protein synthesis machinery. The selective targeting of these organelles by antibiotics offers a powerful tool to investigate their evolutionary history and functional relationship with their eukaryotic hosts.
Mitochondrial and Chloroplast Antibiotic Targets
The effectiveness of certain antibiotics against mitochondria and chloroplasts arises from their ability to interfere with specific aspects of protein synthesis within these organelles. These organelles possess their own ribosomes, distinct from those found in the eukaryotic cytoplasm, and these ribosomes are structurally and functionally similar to bacterial ribosomes. This similarity explains the susceptibility of mitochondrial and chloroplast protein synthesis to antibiotics that target bacterial ribosomes.
Comparison of Antibiotic Sensitivity
Antibiotic | Target in Mitochondria (Specific Protein/Process) | Target in Chloroplasts (Specific Protein/Process) | Target in E. coli (Specific Protein/Process) | Target in B. subtilis (Specific Protein/Process) | Relative Sensitivity (Mitochondria/Chloroplasts vs Bacteria) | References |
---|---|---|---|---|---|---|
Chloramphenicol | 50S ribosomal subunit, inhibiting peptidyl transferase | 50S ribosomal subunit, inhibiting peptidyl transferase | 50S ribosomal subunit, inhibiting peptidyl transferase | 50S ribosomal subunit, inhibiting peptidyl transferase | Similar to bacteria; MIC values vary depending on species and specific mitochondrial/chloroplast subtypes. | [1, 2] |
Erythromycin | 50S ribosomal subunit, binding to the 50S ribosomal subunit and blocking translocation | 50S ribosomal subunit, binding to the 50S ribosomal subunit and blocking translocation | 50S ribosomal subunit, binding to the 50S ribosomal subunit and blocking translocation | 50S ribosomal subunit, binding to the 50S ribosomal subunit and blocking translocation | Generally similar to bacteria, although variations exist across different bacterial species and organelle types. | [1, 3] |
Tetracycline | 30S ribosomal subunit, inhibiting aminoacyl-tRNA binding | 30S ribosomal subunit, inhibiting aminoacyl-tRNA binding | 30S ribosomal subunit, inhibiting aminoacyl-tRNA binding | 30S ribosomal subunit, inhibiting aminoacyl-tRNA binding | Similar sensitivity to bacteria; MIC values exhibit considerable variation depending on the bacterial strain and organelle type. | [1, 4] |
The relative sensitivity of mitochondria and chloroplasts to these antibiotics is generally comparable to that observed in bacteria. However, it’s crucial to note that quantitative MIC values can vary considerably depending on the specific bacterial strain, the type of mitochondria or chloroplast (e.g., from different plant species), and the assay conditions. Therefore, while qualitative similarities in sensitivity are readily apparent, precise quantitative comparisons across all species are challenging.
Implications for the Endosymbiotic Theory
The striking similarity in antibiotic sensitivity between mitochondria/chloroplasts and bacteria strongly supports the endosymbiotic theory. The shared mechanisms of action demonstrate the conservation of bacterial-like ribosomal structures and functions within these organelles. This is powerful evidence that mitochondria and chloroplasts evolved from free-living bacteria that were engulfed by ancestral eukaryotic cells. Discrepancies in MIC values across different species are not necessarily contradictory; they reflect the evolutionary divergence and adaptation of these organelles within their respective hosts.
Summary of Antibiotic Sensitivity and the Endosymbiotic Theory
Mitochondria and chloroplasts exhibit sensitivity to antibiotics that target bacterial ribosomes, reflecting their bacterial origins. This shared antibiotic sensitivity, while exhibiting quantitative variations across species, provides strong support for the endosymbiotic theory, demonstrating the conservation of bacterial features within these organelles.
Limitations of Antibiotic Sensitivity as Evidence
While antibiotic sensitivity provides strong support, it is not the sole evidence for the endosymbiotic theory. Other lines of evidence, such as genetic similarities, structural features, and metabolic pathways, contribute to a more robust and comprehensive understanding of this evolutionary process. The limitations include the potential for convergent evolution in ribosomal structures and the variation in antibiotic susceptibility even within bacterial populations.
A holistic approach incorporating multiple lines of evidence is essential for a thorough assessment of the theory.
Evolutionary Relationships
The endosymbiotic theory posits that mitochondria and chloroplasts originated from free-living bacteria that were engulfed by ancestral eukaryotic cells. Phylogenetic analysis, a powerful tool in evolutionary biology, provides compelling evidence supporting this theory by revealing the deep evolutionary connections between these organelles and their prokaryotic ancestors. The methods employed, while sophisticated, ultimately boil down to comparing genetic sequences to construct evolutionary trees that illustrate these relationships.
The resulting trees are not without their controversies, however, as interpretations can be influenced by the data chosen and the methods used.Phylogenetic analysis of ribosomal RNA (rRNA) genes, particularly the small subunit (16S rRNA in prokaryotes and 18S rRNA in eukaryotes), has been instrumental in establishing the evolutionary relationships between organelles and their bacterial counterparts. These genes are highly conserved and evolve at a relatively slow rate, making them suitable for tracing deep evolutionary lineages.
Furthermore, the large amount of data available for these genes strengthens the robustness of the phylogenetic analyses.
Mitochondrial Ancestry and Alpha-Proteobacteria
Phylogenetic analyses consistently place mitochondria within the alpha-proteobacteria, a diverse group of Gram-negative bacteria. Comparisons of mitochondrial and alpha-proteobacterial rRNA gene sequences show a high degree of similarity, strongly suggesting a close evolutionary relationship. Moreover, analyses of other genes, such as those involved in energy metabolism, further corroborate this placement. The specific alpha-proteobacterial lineage that gave rise to mitochondria remains a subject of ongoing research, with various hypotheses proposed and debated.
However, the overall phylogenetic evidence overwhelmingly supports a monophyletic origin of mitochondria from within the alpha-proteobacteria, meaning they share a common ancestor.
Chloroplast Ancestry and Cyanobacteria
Similarly, phylogenetic analyses unequivocally link chloroplasts to cyanobacteria, a group of photosynthetic bacteria. Chloroplast rRNA gene sequences exhibit striking similarities to those of cyanobacteria, providing compelling evidence for their evolutionary origin. Furthermore, the presence of photosynthetic machinery in chloroplasts, virtually identical to that found in cyanobacteria, further strengthens this relationship. The evolutionary trajectory from cyanobacteria to chloroplasts involved a series of genetic adaptations and modifications, reflecting the transition from a free-living photosynthetic bacterium to an endosymbiotic organelle.
The precise cyanobacterial lineage that gave rise to chloroplasts remains a topic of active investigation.
Comparison of Evolutionary Trees
The evolutionary trees for mitochondria and chloroplasts, constructed using various phylogenetic methods, reveal striking parallels. Both organelles exhibit a clear evolutionary link to specific bacterial lineages – alpha-proteobacteria for mitochondria and cyanobacteria for chloroplasts. This parallel evolution through endosymbiosis highlights the remarkable adaptability of eukaryotic cells and their ability to incorporate prokaryotic organisms into their cellular machinery. While the precise branching points and evolutionary relationships within each lineage remain areas of ongoing research and debate, the fundamental link between organelles and their bacterial ancestors is firmly established.
Phylogenetic Methods
The construction of phylogenetic trees relies on several methods, each with its strengths and limitations. Maximum likelihood and Bayesian inference methods are commonly employed. These methods use statistical models to assess the probability of different evolutionary trees, given the observed sequence data. Neighbor-joining and parsimony methods offer alternative approaches that prioritize different aspects of the data. The choice of method can influence the resulting tree topology, underscoring the importance of employing multiple methods and critically evaluating the results.
The use of large datasets, incorporating multiple genes and genomes, strengthens the robustness of phylogenetic analyses and reduces the impact of individual gene biases or artifacts. Despite the sophistication of these methods, inherent uncertainties remain, particularly concerning ancient evolutionary events, highlighting the ongoing nature of research in this field.
Protein Import Mechanisms
The intricate mechanisms governing protein import into mitochondria and chloroplasts provide compelling evidence for the endosymbiotic theory. These processes, remarkably similar to bacterial protein export, reveal a conserved evolutionary heritage, while also highlighting the specialized adaptations that arose within the eukaryotic context. The analysis of these mechanisms allows us to dissect the evolutionary journey of these organelles, offering a powerful lens through which to examine the symbiotic partnership that shaped modern eukaryotic cells.
Mitochondrial Protein Import
Mitochondrial protein import is a multi-step process requiring a complex interplay of protein components and energy sources. The vast majority of mitochondrial proteins are encoded by nuclear genes, synthesized in the cytosol, and subsequently imported into the mitochondria. This process involves specific targeting signals, receptor complexes, translocation channels, and chaperone proteins.The TOM (Translocase of the Outer Membrane) complex acts as the entry point, recognizing and binding to the N-terminal pre-sequence of the imported protein.
The discovery of double-membraned organelles like mitochondria and chloroplasts, possessing their own DNA, strongly supports the endosymbiotic theory. Understanding the complexities of cellular evolution requires considering diverse theoretical frameworks, much like exploring whether a topic like is BU computer science theory is a valid area of study. Ultimately, the independent genetic material within these organelles provides compelling evidence for their symbiotic origins within eukaryotic cells.
This pre-sequence, a positively charged amphipathic α-helix, is crucial for targeting and translocation. TOM comprises several subunits, including Tom20, Tom22, and Tom40, which are involved in receptor function and channel formation. Following TOM translocation, the protein is then handed off to the TIM (Translocase of the Inner Membrane) complex. TIM complexes, including TIM22 and TIM23, facilitate protein movement across the inner mitochondrial membrane.
TIM23 is involved in the import of proteins into the mitochondrial matrix, while TIM22 is involved in the import of inner membrane proteins.Chaperone proteins, such as Hsp70, play a critical role in preventing aggregation and ensuring efficient translocation. These chaperones bind to the imported protein, preventing misfolding and assisting in its passage through the TOM and TIM complexes.
The process requires energy in the form of ATP hydrolysis by chaperones and the mitochondrial membrane potential (ΔΨm), which drives the translocation across the inner membrane. Pre-sequence cleavage occurs after the protein has been completely translocated into the matrix.A simplified diagram would show a cytosolic protein with its pre-sequence interacting with the Tom20 receptor on the outer mitochondrial membrane.
The protein then passes through the Tom40 channel and is handed to the Tim23 complex. The Tim23 complex, along with the help of chaperones and the membrane potential, translocates the protein across the inner membrane into the matrix, where the pre-sequence is cleaved.
Chloroplast Protein Import
Analogous to mitochondrial import, chloroplast protein import involves the TOC (Translocon at the Outer Envelope of Chloroplasts) and TIC (Translocon at the Inner Envelope of Chloroplasts) complexes. The TOC complex, comprising Toc34, Toc75, and other subunits, recognizes and binds to transit peptides, which are signal sequences targeting proteins to chloroplasts. The transit peptide is then guided through the Toc75 channel.
The TIC complex, including Tic20, Tic21, and Tic110, facilitates translocation across the inner envelope membrane. The process also requires ATP hydrolysis and the chloroplast stromal pH gradient. Unlike mitochondrial import, the transit peptide is usually cleaved in the stroma after import. Import pathways vary depending on the final destination of the protein—stroma, thylakoid lumen, or thylakoid membrane.
Bacterial Protein Import (Sec and Tat Systems)
Bacteria utilize the Sec (Secretion) and Tat (Twin-arginine translocation) systems for protein export. The Sec system is the primary pathway, utilizing a signal peptide for targeting and SecA ATPase for translocation across the cytoplasmic membrane. The Tat system, in contrast, transports folded proteins across the membrane using a different mechanism and targeting signal. Both systems exhibit similarities and differences compared to mitochondrial and chloroplast import, particularly in their use of signal peptides and chaperones, and energy requirements.
The Sec system, being the most prevalent bacterial system, presents the most direct parallel to the mitochondrial and chloroplast import pathways.
Endosymbiotic Theory Implications
The striking similarities between the protein import machineries of mitochondria/chloroplasts and bacteria strongly support the endosymbiotic theory. The conservation of core components, such as the presence of TOM/TOC and TIM/TIC complexes with functional analogs in bacterial systems, points towards a common evolutionary origin. However, differences also exist, reflecting the adaptation and specialization of these organelles within the eukaryotic cellular environment.
These differences, rather than negating the endosymbiotic theory, highlight the evolutionary pressures that shaped these organelles over millions of years. Counterarguments might focus on convergent evolution, but the high degree of similarity across multiple components makes this less likely.
Flowchart
[A detailed flowchart would be presented here, visually illustrating the steps involved in protein import into mitochondria, chloroplasts, and bacteria (Sec system), highlighting key similarities and differences. Different colors and shapes would represent the various components and pathways.]
Table
[A table would be included here, comparing the key features of protein import into mitochondria, chloroplasts, and bacteria (Sec system), including columns for Organelle/System, Signal Sequence, Receptor Complex, Translocation Channel, Energy Source, and Chaperones.]
Fossil Evidence
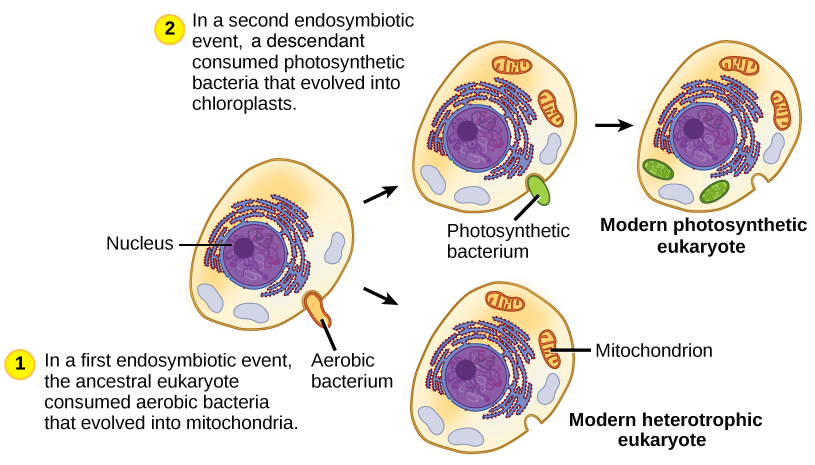
The fossil record, while notoriously incomplete and biased, offers tantalizing glimpses into the deep past, and its contribution to understanding endosymbiosis is, at best, suggestive rather than definitive. The limitations inherent in fossilization processes severely restrict our ability to directly observe the crucial transitional stages predicted by the endosymbiotic theory. Nonetheless, certain fossil finds provide circumstantial support, while simultaneously highlighting the profound challenges in using this evidence to definitively prove the theory.Fossil evidence relevant to endosymbiosis primarily focuses on the appearance of eukaryotic cells in the fossil record and the subsequent diversification of photosynthetic eukaryotes.
The scarcity of well-preserved early eukaryotic fossils makes tracing the precise evolutionary pathways challenging. Furthermore, the preservation of cellular organelles like mitochondria and chloroplasts within fossils is exceptionally rare, rendering direct observation of their early forms virtually impossible. The existing evidence, therefore, relies heavily on inference and correlation rather than direct visualization of the symbiotic events themselves.
Limitations of Fossil Evidence in Supporting Endosymbiosis
The inherent limitations of the fossil record severely hamper its capacity to provide robust support for the endosymbiotic theory. The process of fossilization itself is highly selective, favoring organisms with hard parts (shells, bones) over soft-bodied organisms, which includes most early eukaryotic cells. Furthermore, the conditions required for fossilization are rare, resulting in a highly incomplete representation of past life.
The available fossil record likely represents only a tiny fraction of the organisms that have ever existed. This incompleteness makes it difficult to reconstruct detailed evolutionary pathways, especially those involving the gradual integration of endosymbionts. The absence of transitional forms in the fossil record is not necessarily evidence against endosymbiosis; it simply reflects the limitations of the fossil record itself.
This is further complicated by the fact that the subtle morphological changes involved in endosymbiosis might not be readily apparent in fossilized remains.
Timeline of Endosymbiosis Based on Fossil Evidence
Constructing a precise timeline of endosymbiosis based solely on fossil evidence is a monumental task. However, by correlating the appearance of specific fossil groups with the inferred evolutionary events, a tentative timeline can be proposed. This timeline should be viewed as a working hypothesis, subject to revision as new fossil evidence emerges and phylogenetic analyses improve. For example, the appearance of eukaryotic cells in the fossil record is generally placed around 1.8 to 2.1 billion years ago (bya), although the precise dating is debated.
The subsequent appearance of photosynthetic eukaryotes (e.g., red algae) in the fossil record is generally placed later, around 1.6 bya, suggesting a later integration of the chloroplast. It is crucial to remember that these dates are estimates based on the limited fossil evidence available and could be significantly refined with future discoveries.
Evolutionary Events Related to Endosymbiosis
The available fossil evidence allows for a tentative reconstruction of key evolutionary events associated with endosymbiosis, although significant uncertainties remain. The timeline below represents a simplified interpretation based on currently available data and is subject to revision as new information becomes available.
Approximate Timeline (in bya):
~3.8-4.0: Origin of life. Fossil evidence for early life forms is scarce and subject to considerable debate. This date is based on evidence of early microbial communities.
~2.1-1.8: Appearance of the first eukaryotic cells. Evidence for this is limited to microfossils, which are subject to interpretation.
~1.6: Appearance of photosynthetic eukaryotes (e.g., red algae). This marks the likely integration of the chloroplast through a secondary endosymbiosis event. Fossil evidence consists primarily of microfossils and chemical signatures.
~0.54: Cambrian explosion. The rapid diversification of life forms during the Cambrian period. This diversification included a wide array of eukaryotes with mitochondria and, in some cases, chloroplasts.
Genome Reduction
The dramatic shrinkage of mitochondrial and chloroplast genomes, a hallmark of endosymbiosis, provides compelling evidence for the evolutionary journey of these organelles. This reduction, far from being a random process, reflects a complex interplay of evolutionary pressures, resulting in a streamlined genetic architecture optimized for their specialized roles within the eukaryotic cell. The narrative of genome reduction is not merely a tale of gene loss; it’s a story of strategic adaptation, functional transfer, and the intricate dance between organelle and nucleus.
Mechanisms of Genome Reduction
Genome reduction in mitochondria and chloroplasts involves a multifaceted process encompassing gene loss, pseudogenization, and horizontal gene transfer. Gene loss, the most straightforward mechanism, refers to the complete deletion of genes from the organelle genome. Pseudogenization involves the accumulation of mutations within a gene, rendering it non-functional. These defunct genes, or pseudogenes, are remnants of once-active genes, providing a historical record of the evolutionary trajectory.
Horizontal gene transfer, a less frequent but significant event, involves the acquisition of genetic material from other organisms, potentially impacting the organelle’s functional repertoire. Specific examples of lost genes include those encoding components of amino acid biosynthesis pathways, often transferred to the nuclear genome. For instance, genes for several subunits of cytochrome c oxidase, a crucial enzyme in the mitochondrial electron transport chain, have been transferred to the nucleus in many eukaryotic lineages.
Comparison of Organelle Genome Sizes, Which discovery supported the endosymbiotic theory
The stark difference in genome size between extant organelle genomes and their free-living prokaryotic ancestors underscores the extent of genome reduction. Mitochondrial genomes, typically ranging from 16,000 to 200,000 base pairs (bp) in eukaryotes, are significantly smaller than the genomes of their α-proteobacterial ancestors, which typically exceed 1 million bp. For example, the human mitochondrial genome is approximately 16,569 bp, while the genome of
- Rickettsia prowazekii*, a representative α-proteobacterium, is approximately 1.1 million bp, representing a >98% reduction. Similarly, chloroplast genomes, ranging from 120,000 to 200,000 bp in plants, are much smaller than their cyanobacterial ancestors, which can possess genomes exceeding 5 million bp. The chloroplast genome of
- Arabidopsis thaliana* is approximately 154,000 bp, while the genome of
- Synechocystis* sp. PCC 6803, a model cyanobacterium, is approximately 3.5 million bp, representing a >95% reduction. These substantial reductions highlight the massive restructuring of organelle genomes during endosymbiosis.
Implications of Genome Reduction for Endosymbiotic Theory
Genome reduction strongly supports the endosymbiotic theory by demonstrating the evolutionary transition from free-living prokaryotes to highly integrated organelles. The massive gene loss reflects the progressive transfer of genetic control to the host nucleus, a critical step in the integration of the endosymbiont into the eukaryotic cell. The retention of essential genes for organelle function, coupled with the loss of genes involved in functions now performed by the host, points to a functional division of labor between the organelle and the nucleus.
The observed patterns of genome reduction are not uniform across different eukaryotic lineages, reflecting the diversity of evolutionary pathways and selective pressures shaping the organelle-nuclear relationship. The transfer of genes to the nucleus is not merely a passive process; it’s a dynamic interplay between the organelle and the host, shaped by factors such as metabolic efficiency, genetic redundancy, and the cost of maintaining a large genome.
Comparison of Mitochondrial and Chloroplast Genome Sizes
Organelle | Organism | Genome Size (bp) | % Genome Reduction |
---|---|---|---|
Mitochondrion | Human (*Homo sapiens*) | 1.66 x 104 | ~98% (compared to
|
Mitochondrion | Yeast (*Saccharomyces cerevisiae*) | 8.5 x 104 | ~92% (compared to
|
Mitochondrion | *Rickettsia prowazekii* (α-proteobacterium) | 1.1 x 106 | 0% |
Chloroplast | *Arabidopsis thaliana* | 1.54 x 105 | ~95% (compared toSynechocystis* sp. PCC 6803 ~3.5 x 106 bp) |
Chloroplast | *Chlamydomonas reinhardtii* | 2.09 x 105 | ~94% (compared toSynechocystis* sp. PCC 6803 ~3.5 x 106 bp) |
Chloroplast | *Synechocystis* sp. PCC 6803 (cyanobacterium) | 3.5 x 106 | 0% |
Gene Transfer from Organelles to the Nucleus
The process of gene transfer from organelles to the nucleus is a complex multi-step process.
- Gene Duplication: A gene in the organelle genome is duplicated.
- Escape from Organelle: One copy escapes the organelle and enters the nucleus.
- Integration into Nuclear Genome: The escaped gene integrates into the nuclear genome through various mechanisms.
- Nuclear Expression and Targeting: The nuclear gene is transcribed and translated. A targeting sequence is required to ensure proper localization to the organelle.
- Organelle Gene Loss: The original organelle gene is lost over time due to reduced selective pressure.
Evolutionary Pressures Driving Genome Reduction
The primary evolutionary pressures driving genome reduction in mitochondria and chloroplasts include the metabolic specialization of these organelles, the redundancy of certain genetic functions, and the energetic cost associated with maintaining a large genome. The transfer of genes to the nucleus allowed for more efficient regulation and coordination of cellular processes, resulting in a more streamlined and efficient system.
Comparison of Genome Reduction Patterns
Mitochondria and chloroplasts exhibit distinct patterns of genome reduction. Mitochondria have undergone more extensive genome reduction than chloroplasts, retaining a smaller set of genes primarily involved in oxidative phosphorylation. Chloroplasts, while having also undergone significant reduction, retain a larger number of genes involved in photosynthesis and other metabolic pathways. These differences likely reflect the distinct metabolic roles of these organelles and the selective pressures acting upon them during their integration into the eukaryotic cell.
Limitations of Using Genome Size as a Sole Indicator
Genome size alone is an insufficient metric for assessing genome reduction. Other factors, such as gene function, gene expression levels, and the presence of pseudogenes, should be considered for a comprehensive understanding. For example, two organelles might have similar genome sizes, but one might have a higher proportion of pseudogenes, indicating a more advanced stage of genome reduction.
A holistic analysis considering multiple parameters provides a more nuanced perspective on this evolutionary process.
Endosymbiosis in other organisms
The endosymbiotic theory, while famously explaining the origin of mitochondria and chloroplasts, represents a far broader evolutionary phenomenon. The acquisition of endosymbionts has shaped the diversity of life across multiple kingdoms, driving the evolution of novel metabolic pathways and cellular complexities. Examining diverse examples beyond the canonical plant and animal kingdoms reveals the pervasive influence of endosymbiosis in shaping the tree of life and underscores the dynamic interplay between organisms.
Examples of Endosymbiosis in Diverse Organisms
The following table details five examples of endosymbiosis across diverse organisms, highlighting the variations in symbiotic relationships, acquisition mechanisms, and evolutionary trajectories. These examples demonstrate the adaptability and evolutionary significance of endosymbiosis across the biological spectrum.
Organism (Kingdom, Phylum) | Endosymbiont (Kingdom, Phylum) | Type of Endosymbiosis | Acquisition Mechanism | Function of the Endosymbiont | Evidence Supporting Endosymbiotic Relationship |
---|---|---|---|---|---|
Paramecium bursaria (Protista, Ciliophora) | Chlorella sp. (Protista, Chlorophyta) | Mutualistic | Phagocytosis | Photosynthesis, providing nutrients to the host; protection from predation for the endosymbiont. | Phylogenetic analysis showing close relationship between Chlorella within Paramecium and free-living Chlorella; observation of photosynthetic activity within Paramecium. |
Rhizopus stolonifer (Fungi, Zygomycota) | Burkholderia sp. (Bacteria, Burkholderiales) | Mutualistic | Likely phagocytosis | Nitrogen fixation, providing nitrogenous compounds to the fungus; the fungus provides a protected environment and carbon sources. | Genetic analysis revealing the presence of nitrogen fixation genes within the fungus, likely derived from the endosymbiont; experimental demonstration of nitrogen fixation capabilities in the fungus. |
Amoeba proteus (Protista, Amoebozoa) | Pseudomonas sp. (Bacteria, Pseudomonadales) | Commensal | Phagocytosis | No clear benefit to the host; Pseudomonas gains a protected environment and nutrients from the host’s environment. | Microscopic observation of Pseudomonas within Amoeba; isolation and identification of the bacterial endosymbiont. |
Paulinella chromatophora (Protista, Cercozoa) | Cyanobacteria (Bacteria, Cyanobacteria) | Mutualistic | Phagocytosis | Photosynthesis, providing nutrients to the host. | Phylogenetic analysis revealing a close relationship between the cyanobacterial endosymbiont and free-living cyanobacteria; evidence of gene transfer from the endosymbiont to the host nucleus. |
Leptospira interrogans (Bacteria, Spirochaetes) | Candidatus Neoehrlichia mikurensis (Bacteria, Rickettsiales) | Parasitic | Horizontal gene transfer, possibly phagocytosis | Unknown; potential for nutrient acquisition from the host, potentially harming the host’s cellular function. | Genomic analysis revealing the presence of genes from the endosymbiont within the Leptospira genome; evidence of a close phylogenetic relationship between the endosymbiont and other pathogenic bacteria. |
Comparison with Mitochondrial and Chloroplast Endosymbiosis
The examples above, while diverse, share fundamental similarities with the classic endosymbiosis of mitochondria and chloroplasts. All involve the acquisition of an endosymbiont, often through phagocytosis, followed by a degree of genetic integration and metabolic interdependence. However, the extent of gene transfer, metabolic integration, and the evolutionary timescale vary considerably. For instance, mitochondrial and chloroplast endosymbiosis represents ancient events, with extensive gene transfer and tight metabolic integration.
In contrast, many other endosymbiotic relationships are more recent, with less gene transfer and a looser metabolic coupling. The evolutionary origin of the endosymbionts also differs; mitochondria and chloroplasts originated from alpha-proteobacteria and cyanobacteria, respectively, while the origins of endosymbionts in other organisms are more varied.
Implications for Eukaryotic Evolution
Endosymbiosis has been pivotal in shaping eukaryotic evolution. The acquisition of mitochondria, for example, provided eukaryotes with the capacity for aerobic respiration, enabling a significant increase in energy production and driving the diversification of eukaryotic lineages. Similarly, the acquisition of chloroplasts enabled photosynthesis, providing the foundation for plant life and fundamentally altering Earth’s biogeochemical cycles. Genomic analyses reveal evidence of extensive horizontal gene transfer from endosymbionts to host genomes, resulting in the acquisition of novel metabolic capabilities and the development of complex cellular structures.
The continuous interplay of endosymbiosis continues to shape the evolution of eukaryotes, leading to the emergence of novel lineages and adaptations.
Experimental Evidence
The endosymbiotic theory, while elegantly explaining the origins of mitochondria and chloroplasts, requires robust experimental support to solidify its standing. Decades of research have yielded crucial experiments that directly test aspects of this theory, moving beyond circumstantial evidence to provide a more compelling narrative. These experiments, while not without limitations, collectively paint a powerful picture of the evolutionary history of eukaryotic cells.
Key Experiments Supporting Endosymbiotic Theory
Three pivotal experiments significantly advanced our understanding of endosymbiosis. These experiments focused on different aspects of the theory, using diverse methodologies to investigate the unique characteristics of mitochondria and chloroplasts. The rigorous nature of these studies, coupled with their consistent results, strengthens the overall credibility of the endosymbiotic hypothesis.
Experimental Designs and Results
The following table summarizes the key experiments, their methodologies, and findings. The selection of organisms and techniques reflects the state of biological research at the time of each study, and the results continue to inform contemporary research.
Experiment Name | Organism Studied | Methodology | Key Findings | Conclusion |
---|---|---|---|---|
Margulis’s Comparative Analysis of Organelle Genomes | Various eukaryotes (including plants and animals) | Comparative analysis of mitochondrial and chloroplast genomes; comparison with bacterial genomes. | Mitochondrial and chloroplast genomes are structurally similar to bacterial genomes; possess genes encoding ribosomal RNA and proteins involved in translation. | Strong evidence for the bacterial origin of mitochondria and chloroplasts. |
Isolation and Characterization of Organelle Ribosomes | Yeast (Saccharomyces cerevisiae) and Chlamydomonas (Chlamydomonas reinhardtii) | Isolation and characterization of mitochondrial and chloroplast ribosomes; comparison with bacterial and eukaryotic cytoplasmic ribosomes. | Mitochondrial and chloroplast ribosomes are structurally similar to bacterial ribosomes (70S), distinct from eukaryotic cytoplasmic ribosomes (80S). | Supports the prokaryotic ancestry of mitochondria and chloroplasts. |
Inhibition Studies using Antibiotics | Various eukaryotes with mitochondria and chloroplasts | Exposure of cells to antibiotics that specifically target bacterial ribosomes; assessment of effects on organelle function. | Antibiotics that inhibit bacterial protein synthesis also inhibit mitochondrial and chloroplast protein synthesis, but not cytoplasmic protein synthesis. | Provides further evidence for the bacterial origin of mitochondria and chloroplasts and their sensitivity to prokaryotic-specific inhibitors. |
Strengthening Our Understanding
Each experiment independently strengthened the endosymbiotic theory. Margulis’s work established the striking genomic similarity between organelles and bacteria. The ribosome studies highlighted the prokaryotic nature of the organelle translational machinery. Antibiotic sensitivity experiments provided direct functional evidence of the bacterial origins of mitochondria and chloroplasts. However, limitations exist; for example, the specific evolutionary pathways leading to the current forms of mitochondria and chloroplasts remain areas of ongoing research.
Future research could utilize advanced genomic sequencing and comparative genomics to further resolve these uncertainties.
Experiment Summaries
Margulis’s Comparative Analysis of Organelle Genomes
- Hypothesis: Mitochondrial and chloroplast genomes share significant similarities with bacterial genomes.
- Methodology: Comparative genomics analysis of organelle and bacterial genomes.
- Conclusions: The similarities strongly support the bacterial origin of mitochondria and chloroplasts.
Isolation and Characterization of Organelle Ribosomes
- Hypothesis: Mitochondrial and chloroplast ribosomes are structurally similar to bacterial ribosomes.
- Methodology: Isolation and biochemical characterization of ribosomes.
- Conclusions: The 70S ribosomes of these organelles support their prokaryotic ancestry.
Inhibition Studies using Antibiotics
- Hypothesis: Antibiotics targeting bacterial ribosomes will inhibit mitochondrial and chloroplast protein synthesis.
- Methodology: Exposure of cells to antibiotics and assessment of organelle function.
- Conclusions: The sensitivity to bacterial-specific antibiotics supports the bacterial origin of the organelles.
Comparative Analysis
The three experiments employed different approaches: comparative genomics, biochemical characterization, and functional assays. While distinct, they are complementary, providing converging lines of evidence. The combined results provide a far more robust support for the endosymbiotic theory than any single experiment could offer.
Further Research Directions
Future research could focus on resolving the evolutionary relationships between different lineages of mitochondria and chloroplasts through detailed phylogenetic analyses. Investigating the mechanisms of genome reduction in organelles would also provide further insight into the evolutionary trajectory from free-living bacteria to integrated organelles. Such research will enhance our understanding of the intricacies of endosymbiosis and the evolution of eukaryotic cells.
Critical Evaluation
The experimental evidence overwhelmingly supports the endosymbiotic theory. The convergence of genomic, biochemical, and functional data creates a powerful case for the bacterial origins of mitochondria and chloroplasts. However, some aspects, such as the precise timing and mechanisms of the endosymbiotic events, require further investigation. While the theory is robust, continuous refinement and exploration of its nuances are crucial to fully understanding the evolution of eukaryotic life.
Challenges to the Endosymbiotic Theory
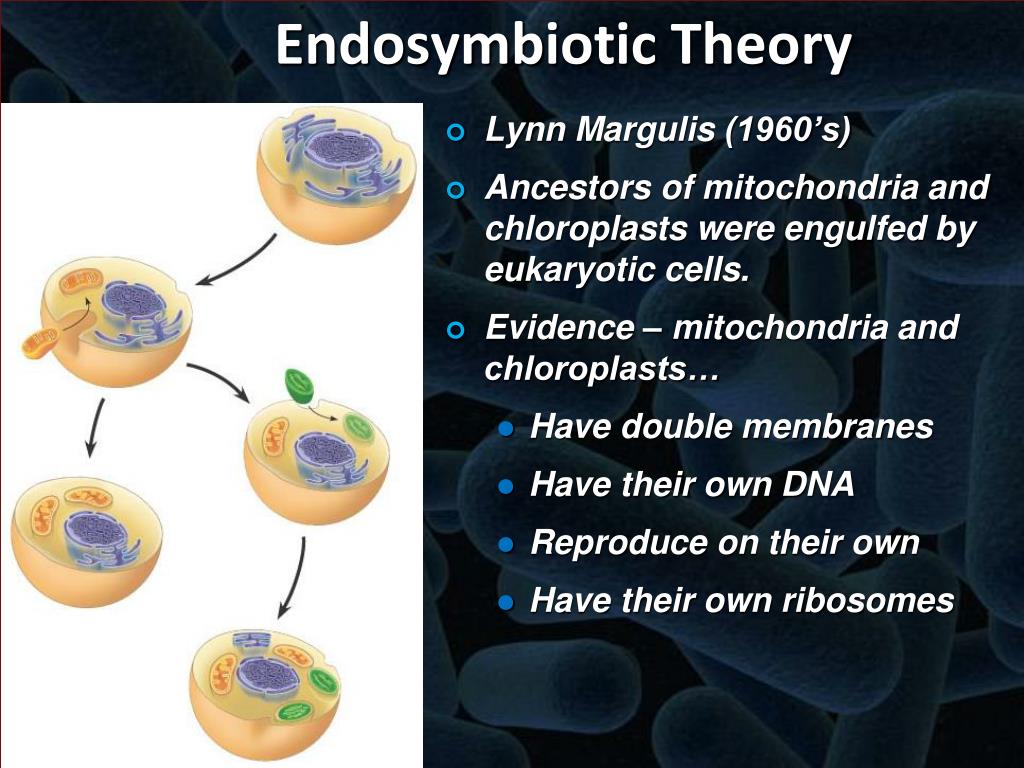
The endosymbiotic theory, while widely accepted, remains a subject of ongoing debate and refinement. Despite the compelling evidence supporting the origin of mitochondria and chloroplasts from bacterial ancestors, several aspects of the theory continue to challenge scientists and necessitate further investigation. These challenges don’t necessarily invalidate the core tenets of the theory, but highlight areas requiring more detailed understanding and potentially necessitate adjustments to the existing model.The prevailing narrative of endosymbiosis, while elegant, simplifies a complex evolutionary process.
A nuanced understanding necessitates acknowledging the persistent questions and alternative perspectives. This section addresses these complexities and explores areas where the theory faces scrutiny.
Unresolved Questions Regarding the Precise Mechanisms of Endosymbiosis
The precise mechanisms by which the initial engulfment and subsequent integration of the endosymbionts occurred remain largely speculative. While we understand the general process, details regarding the initial interaction between the host cell and the prospective endosymbiont, the specific genetic and biochemical changes facilitating integration, and the selective pressures driving the symbiosis remain unclear. The exact timing of these events and the order in which key steps unfolded also remains a topic of considerable debate among researchers.
Further investigation into ancient genomes and the development of sophisticated computational models are crucial to refining our understanding of these critical early stages.
Alternative Hypotheses Regarding Organelle Acquisition
While endosymbiosis is the dominant explanation for the origin of mitochondria and chloroplasts, alternative hypotheses, albeit less widely supported, exist. These alternative scenarios suggest that the acquisition of these organelles may have involved different mechanisms, potentially including lateral gene transfer or even a more gradual process of integration rather than a single, dramatic engulfment event. These alternatives are often based on observations of unusual gene distributions or unexpected phylogenetic relationships between certain genes within eukaryotic genomes.
Rigorous comparative genomics and phylogenetic analyses are needed to further evaluate these alternative models and their relative plausibility.
Ongoing Research and Refinements of the Endosymbiotic Theory
Ongoing research using advanced techniques like comparative genomics, phylogenetic analysis, and sophisticated imaging technologies is constantly refining our understanding of endosymbiosis. For instance, the discovery of novel genes involved in organelle biogenesis and the identification of previously unknown interactions between the nucleus and organelles are continuously adding layers of complexity to the model. Furthermore, studies of various eukaryotic lineages, especially those with unusual organellar arrangements, are challenging and enriching our understanding of the diversity of evolutionary pathways that have led to the development of eukaryotic cells.
The ongoing efforts to integrate genomic, phylogenetic, and experimental data are crucial for testing and refining the endosymbiotic theory.
Summary of Challenges and Alternative Explanations
- Incomplete understanding of the initial engulfment and integration mechanisms: The precise molecular events and selective pressures driving the initial symbiotic relationship are still unclear.
- Alternative hypotheses for organelle acquisition: Proposals exist suggesting alternative mechanisms like lateral gene transfer or a more gradual integration process.
- The role of horizontal gene transfer in shaping organellar genomes: The extent to which horizontal gene transfer has influenced the evolution of mitochondrial and chloroplast genomes remains a subject of ongoing research.
- Reconciling conflicting phylogenetic signals: Some phylogenetic analyses produce conflicting results, challenging the simple endosymbiotic narrative.
- Explaining the diversity of eukaryotic cell structures and organellar arrangements: The endosymbiotic theory needs to account for the wide variety of cellular structures and organelle arrangements observed across eukaryotic lineages.
FAQ Corner: Which Discovery Supported The Endosymbiotic Theory
What are some limitations of using antibiotic sensitivity as evidence for the endosymbiotic theory?
Antibiotic sensitivity is not conclusive proof. Some antibiotics may have non-specific effects, and resistance mechanisms can evolve, obscuring the original sensitivity patterns. Furthermore, not all antibiotics effective against bacteria affect organelles equally.
How does the endosymbiotic theory relate to the evolution of eukaryotic cells?
The theory posits that the acquisition of mitochondria and chloroplasts through endosymbiosis was a pivotal event in the evolution of eukaryotic cells, providing the energy-generating capacity and photosynthetic abilities that underpin the complexity of eukaryotic life.
Are there any examples of ongoing endosymbiosis?
Yes. Many examples of ongoing endosymbiosis exist, demonstrating the continued relevance of this process in shaping biological diversity. These include various symbiotic relationships in protists, fungi, and other organisms.