What does the place theory of pitch perception suggest? This question delves into a fundamental aspect of auditory neuroscience, exploring how our brains decipher the complex world of sound. The theory proposes that our perception of pitch is directly linked to the specific location on the basilar membrane within the cochlea that is maximally stimulated by a given frequency.
This elegant model, developed over decades of research, provides a compelling framework for understanding how we distinguish between high and low notes. However, as we’ll explore, the theory has limitations and requires consideration alongside other models of pitch perception to provide a complete picture.
The journey to understanding pitch perception begins with the intricate structure of the inner ear. Sound waves traveling through the ear canal cause vibrations in the basilar membrane, a crucial component of the cochlea. Different frequencies stimulate different locations along this membrane, creating a tonotopic map. Hair cells, located on the basilar membrane, convert these mechanical vibrations into electrical signals, which are then transmitted to the brain via the auditory nerve.
The brain interprets the location of these activated hair cells as a specific pitch. This process, while seemingly straightforward, involves complex interactions between different cell types, neural pathways, and cortical processing.
Introduction to Place Theory
The world of sound perception is a fascinating labyrinth, and one of the key players in unraveling its mysteries is the place theory of pitch perception. This theory, a cornerstone of auditory neuroscience, elegantly explains how we distinguish between high and low-pitched sounds, a feat that allows us to appreciate the soaring melodies of an opera and the rumbling bass of a rock concert with equal aplomb.
It’s a theory so elegant, it almost makes one want to burst into song – but let’s restrain ourselves for the sake of scientific rigor.Place theory proposes that the perception of pitch is directly related to the location on the basilar membrane of the cochlea where maximum vibration occurs. Imagine the cochlea, that spiraled marvel of the inner ear, as a finely tuned piano keyboard.
Different frequencies of sound cause maximum displacement at different points along this “keyboard,” triggering the firing of specific hair cells in those precise locations. High-frequency sounds stimulate hair cells near the base of the cochlea, while low-frequency sounds activate hair cells closer to the apex. This spatial coding of frequency is the heart of place theory.
Historical Development of Place Theory
The development of place theory wasn’t a sudden “eureka!” moment, but rather a gradual refinement of ideas, built upon the work of several brilliant minds. Hermann von Helmholtz, a polymath whose contributions extended far beyond auditory perception, proposed a version of place theory in the 19th century. His work, while insightful, lacked the precise understanding of the cochlea’s mechanics that later research would provide.
Subsequent research, employing advanced techniques like microelectrode recordings, provided crucial experimental support, refining and strengthening Helmholtz’s original insights. These experiments directly measured the activity of individual hair cells in response to different frequencies, providing concrete evidence for the spatial coding of frequency. The contributions of researchers like Georg von Békésy, who won a Nobel Prize for his work on the mechanics of the cochlea, were instrumental in solidifying the place theory’s position as a central tenet of auditory perception.
He meticulously studied the movement of the basilar membrane in response to sound stimuli, providing a detailed understanding of the resonant properties of this crucial structure.
Place Theory and Pitch Perception: A Concise Definition
Place theory, in its simplest form, posits that our brains decode the frequency of a sound by identifying the location along the basilar membrane where the sound wave causes the greatest displacement. High frequencies cause maximal displacement near the base, while low frequencies trigger maximal displacement closer to the apex. This location is then translated into a perceived pitch.
This elegant mechanism allows us to discriminate between a wide range of frequencies, transforming the complex vibrations of sound waves into the rich tapestry of auditory experience. The precision of this mechanism is remarkable, allowing us to discern subtle differences in pitch, even within complex soundscapes.
Basilar Membrane and Frequency Encoding
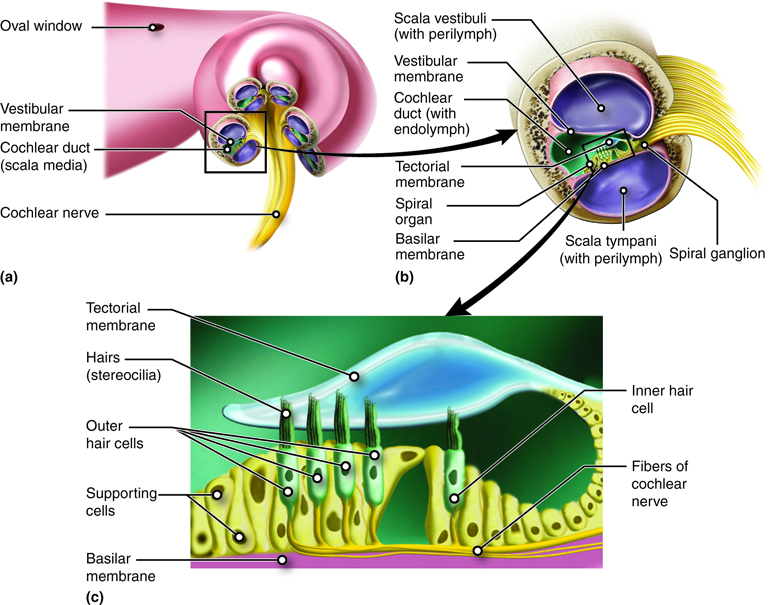
Prepare yourself for a thrilling journey into the inner ear, where the seemingly mundane act of hearing transforms into a symphony of intricate biological mechanisms! We’ll be exploring the basilar membrane, a structure so crucial to our perception of sound that its absence would leave us living in a world of silent, auditory nothingness (a terrifying prospect, wouldn’t you agree?).The basilar membrane, residing within the cochlea – that spiraling, snail-shell-shaped marvel of the inner ear – is a thin, flexible strip of tissue.
Imagine it as a miniature, exquisitely sensitive harp, with each “string” responding to a specific range of sound frequencies. This isn’t just any harp, mind you; it’s a bio-engineered masterpiece, capable of discriminating between the delicate tinkling of a crystal glass and the thunderous roar of a rock concert. Its remarkable sensitivity allows us to perceive a vast range of sounds, from the gentle whisper of a lover to the deafening shriek of a jet engine.
Basilar Membrane Structure and Function
The basilar membrane is wider and more flexible at its apex (the tip of the cochlea) and narrower and stiffer at its base (near the oval window where sound enters). This graded stiffness is the key to its frequency-encoding abilities. Think of it like a trampoline: the wider, more flexible end vibrates most readily to low-frequency sounds (like the deep rumble of a bass guitar), while the narrower, stiffer base responds best to high-frequency sounds (like the piercing shriek of a whistle).
This differential response is what allows us to distinguish between the low notes of a cello and the high notes of a flute. The mechanical vibrations of the basilar membrane then trigger the hair cells, initiating the process of auditory transduction and sending the neural signals to the brain for interpretation. It’s a marvel of engineering, really, far surpassing anything we could currently create artificially.
Frequency-Specific Stimulation of the Basilar Membrane
Different frequencies of sound cause maximal vibration at different locations along the basilar membrane. High-frequency sounds cause maximal displacement near the base, which is stiff and narrow. Low-frequency sounds, on the other hand, create maximal displacement near the apex, which is wide and flexible. This tonotopic organization – the systematic mapping of frequencies to locations – is fundamental to place theory.
The brain interprets the location of maximal stimulation as the perceived pitch. It’s like a highly specialized map, where each point corresponds to a specific frequency, allowing our brains to decipher the complex world of sound. It’s rather like a sophisticated postal service, delivering frequency information to the correct location in the brain with impressive accuracy and speed.
Frequency and Location of Maximal Stimulation
This table illustrates the relationship between frequency and the location of maximal stimulation on the basilar membrane. Remember, these are approximate values, as the exact location varies slightly between individuals and depends on various factors. But it gives you a general idea of the tonotopic map’s remarkable precision.
Frequency (Hz) | Location on Basilar Membrane | Approximate Distance from Base (mm) | Corresponding Sound Example |
---|---|---|---|
200 | Apex (tip of cochlea) | 35 | Low-pitched bass drum |
1000 | Mid-cochlea | 15 | Human voice (mid-range) |
4000 | Base (near oval window) | 5 | High-pitched whistle |
15000 | Very near base | 1 | High-frequency squeak |
Hair Cells and Neural Transduction
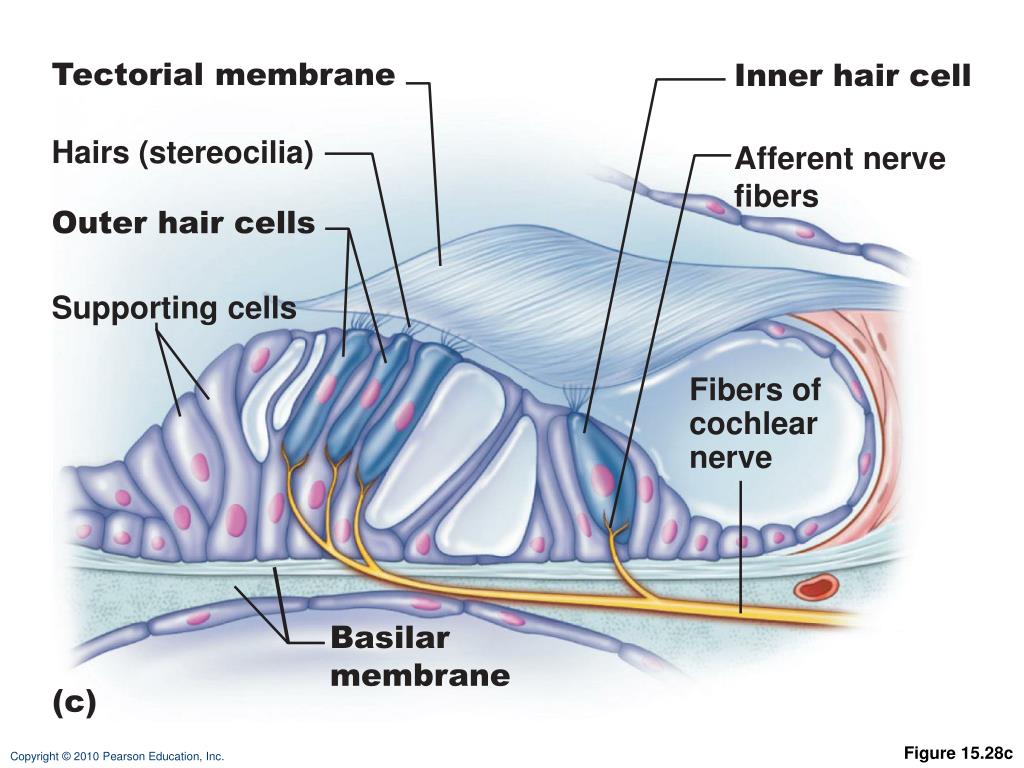
The seemingly simple act of hearing involves a breathtakingly complex interplay of mechanical vibrations, delicate cellular structures, and sophisticated neural processing. We’ve already explored the groundwork laid by the basilar membrane’s frequency-dependent response, but the real magic happens at the level of the hair cells – the tiny, exquisitely sensitive transducers that convert sound waves into electrical signals the brain can understand.
Prepare yourself for a microscopic adventure into the world of auditory perception!
Mechanoelectrical Transduction in Hair Cells
Hair cells, the unsung heroes of our auditory system, are remarkable cells that perform the crucial task of converting mechanical energy (sound vibrations) into electrical signals. This process, known as mechanoelectrical transduction, is orchestrated by a fascinating arrangement of stereocilia, tip links, and ion channels. Imagine a hair cell as a miniature, exquisitely sensitive microphone, capturing the faintest whispers of sound.
A single hair cell possesses numerous stereocilia, tiny hair-like projections arranged in a precise order. These stereocilia are connected by delicate protein filaments called tip links, which act as the crucial link between mechanical movement and electrical signaling. When sound waves cause the basilar membrane to vibrate, the stereocilia are deflected. This deflection stretches the tip links, opening mechanically gated ion channels.
The influx of positively charged ions, primarily potassium (K+) and calcium (Ca2+), depolarizes the hair cell membrane, triggering a cascade of electrical events that ultimately lead to the release of neurotransmitters and the generation of nerve impulses. The hair cell, having successfully converted a mechanical stimulus into an electrical one, sends this message along the auditory nerve to the brain for interpretation.
Okay, so place theory’s all about how different sound frequencies activate different parts of your inner ear, right? It’s kinda like how choosing the right tint for a photo, check out this awesome guide how to tint photos correctly color theory , depends on understanding color theory. Basically, both are about specific locations causing specific effects – frequencies for hearing and colors for vision, totally rad, right?
A simplified diagram would show a hair cell with its bundle of stereocilia, the tip links connecting adjacent stereocilia, and ion channels embedded in the cell membrane. The deflection of stereocilia in one direction opens the channels, while deflection in the opposite direction closes them.
Types of Hair Cells and Their Functions
Within the organ of Corti, two distinct types of hair cells play crucial, yet different, roles: inner hair cells (IHCs) and outer hair cells (OHCs). These cells, while both involved in hearing, have strikingly different structural and functional characteristics. Think of them as a highly specialized team, each member contributing unique skills to the overall auditory experience. The table below summarizes their key differences:
Feature | Inner Hair Cells (IHCs) | Outer Hair Cells (OHCs) |
---|---|---|
Primary Function | Auditory signal transduction | Amplification of auditory signals |
Stereocilia | One row, shorter | Three rows, longer |
Afferent Innervation | Primarily afferent, many synapses per cell | Fewer afferent synapses per cell |
Efferent Innervation | Less efferent innervation | Extensive efferent innervation |
Role in Hearing | Primary sensory transduction for hearing | Amplification and fine-tuning of auditory signals |
Supporting cells within the organ of Corti provide structural support and maintain the optimal environment for hair cell function. They act as a vital infrastructure, ensuring the hair cells can operate at peak efficiency. These supporting cells are essential for the overall health and function of the organ of Corti.
Pitch Perception and Hair Cell Location
The cochlea isn’t just a passive receiver of sound; it’s a marvel of tonotopic organization, meaning different frequencies stimulate different regions along its length. This spatial arrangement is the key to our ability to distinguish between high and low pitches. Imagine the cochlea as a piano keyboard, with each key representing a specific frequency, and each hair cell responding to a specific key.
The basilar membrane, with its varying stiffness and width along its length, plays a crucial role in this frequency analysis. A diagram depicting the tonotopic map would illustrate the systematic arrangement of hair cells along the basilar membrane, with high-frequency sounds activating hair cells near the base (the stiff end) and low-frequency sounds activating hair cells near the apex (the floppy end).
The brain then interprets the location of activated hair cells to determine the pitch of the sound. The auditory pathways, a complex network of neural connections, transmit this information to the auditory cortex for further processing and interpretation.
Hair Cell Damage and Hearing Loss
Exposure to loud noises, the relentless march of time (age-related hearing loss), and certain diseases can all lead to hair cell damage. This damage disrupts the delicate process of mechanoelectrical transduction, resulting in hearing impairment. The degree of hearing loss depends on the extent and location of the damage. For instance, damage to hair cells responsible for high-frequency sounds can lead to difficulty hearing high-pitched sounds, while widespread damage results in significant hearing loss across the frequency spectrum.
Hair Cell Regeneration
The prospect of hair cell regeneration offers a beacon of hope for restoring hearing function in individuals with hearing loss. Research is actively exploring strategies to stimulate the regeneration of hair cells, including gene therapy and the use of stem cells. While significant challenges remain, the potential for restoring hearing through hair cell regeneration is a powerful driver of ongoing research efforts.
Limitations of Place Theory
Ah, the place theory of pitch perception – a beautiful idea, really. Like a finely tuned orchestra, it suggests that different locations on the basilar membrane vibrate to different frequencies, creating a neat map of sound. But, as with most elegant theories, reality throws a few curveballs. Let’s explore where this theory falls a bit flat, shall we?The place theory, while wonderfully intuitive, doesn’t quite hit all the right notes, particularly in the lower registers.
Its accuracy in predicting pitch perception is primarily limited to higher frequencies, generally above 500 Hz. Below this frequency range, things get a bit muddier.
Low-Frequency Sound Perception and Place Theory’s Shortcomings
For low-frequency sounds, the vibrations on the basilar membrane become less localized. Imagine trying to pinpoint the exact location of a gentle ripple in a large pond – it’s difficult, right? Similarly, low-frequency sounds cause a broader area of the basilar membrane to vibrate, making it challenging to precisely determine the frequency based solely on the location of maximum displacement.
This lack of precise localization hinders the place theory’s ability to accurately explain our perception of low-pitched sounds like a rumbling bass guitar or a deep male voice. These sounds, despite their clear pitch to us, create a fuzzy map on the basilar membrane, challenging the place theory’s crisp, location-based model. Think of it like trying to use a magnifying glass to read a newspaper printed in tiny, blurry font; the detail just isn’t there.
Comparison of Place Theory and Temporal Theory
Place theory, with its neat spatial mapping, finds itself in a friendly competition with temporal theory. Temporal theory, the underdog, proposes that pitch perception is encoded by the timing of neural firing patterns. Imagine a drummer keeping time – the consistent beat, even if not precisely located, tells you the rhythm. Similarly, temporal theory suggests that the rate of neural impulses mirrors the frequency of the sound.
While place theory excels in higher frequencies, temporal theory shines in lower frequencies where the neural firing rates can reliably reflect the sound’s frequency. It’s not a case of one theory being definitively “better,” but rather that they work in concert, like a two-part harmony, to explain the full range of pitch perception. Place theory provides the high-frequency clarity, while temporal theory adds the low-frequency depth.
The two approaches, while distinct, offer complementary explanations for the complexity of our auditory experience.
Neural Pathways and Pitch Processing
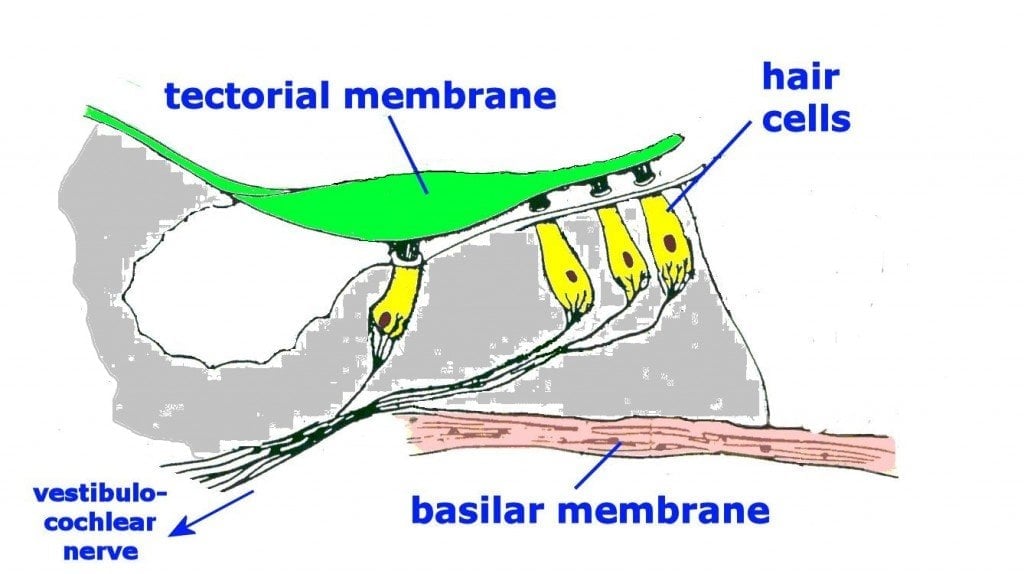
The journey of a sound wave, from the delightful tinkle of a dropped teacup to the ear-splitting roar of a rock concert, is a surprisingly complex adventure. Once the mechanical dance of the basilar membrane has been completed, the real party begins – the neural transmission of auditory information. This is where the brain, that magnificent organ of interpretation, steps in to decipher the symphony of sounds.
Think of it as a high-speed auditory express train, delivering crucial information about pitch, loudness, and timbre to the central station of consciousness.The auditory information, encoded in the patterns of neural firing from the cochlea, embarks on a fascinating journey to the brain. This is not a single, straight path, but rather a network of intricately connected highways and byways.
The story begins with the spiral ganglion neurons, whose axons converge to form the auditory nerve (also known as the vestibulocochlear nerve, because it’s a bit of a multi-tasker). This nerve carries the auditory signals to the brainstem, specifically the cochlear nuclei. From there, the information travels along a series of relay stations, including the superior olivary complex (which is particularly important for sound localization – knowing where the sound is coming from), the lateral lemniscus, and the inferior colliculus.
Finally, the information arrives at the medial geniculate nucleus (MGN) of the thalamus, acting as a final checkpoint before reaching the auditory cortex. It’s quite the auditory odyssey!
Auditory Pathways and Spatial Information Processing
The brain doesn’t just passively receive information; it actively processes it. In the context of place theory, the brain interprets pitch by decoding the location of maximal stimulation along the basilar membrane. Remember that different frequencies stimulate different areas of the basilar membrane? Well, the brain cleverly maps this spatial information onto a tonotopic organization within the auditory pathways.
This means that neurons responding to similar frequencies tend to be clustered together. This tonotopic organization is maintained throughout the auditory pathways, from the cochlea to the auditory cortex, allowing the brain to create a “map” of sound frequencies. Imagine it as a highly organized neighborhood where neurons specializing in high-pitched sounds live next to each other, and those who prefer low-pitched sounds have their own distinct section.
It’s a remarkably efficient system.
Flowchart Illustrating Neural Pathways in Pitch Perception (Place Theory)
The following flowchart illustrates the journey of auditory information according to the place theory, highlighting the key neural structures and their roles. The beauty of a flowchart is its ability to visually represent the complexity of these processes, making the intricate journey of sound perception more accessible. Think of it as a map to the brain’s auditory processing center![Imagine a flowchart here.
It would start with the Cochlea, showing hair cells stimulated at different locations depending on frequency. Arrows would lead to the Spiral Ganglion, then to the Cochlear Nuclei, Superior Olivary Complex, Lateral Lemniscus, Inferior Colliculus, Medial Geniculate Nucleus (MGN) of the Thalamus, and finally the Auditory Cortex. Each stage would indicate the tonotopic organization of the neural signals, showing how the spatial information from the basilar membrane is preserved throughout the pathway.
The final stage in the Auditory Cortex would show the interpretation of pitch based on the location of activation.]
Evidence Supporting Place Theory: What Does The Place Theory Of Pitch Perception Suggest
The place theory of pitch perception, while seemingly straightforward – higher frequencies activate hair cells closer to the base of the basilar membrane, lower frequencies closer to the apex – requires robust experimental support to solidify its position as a cornerstone of auditory neuroscience. Fortunately, a wealth of evidence, from ingenious experiments to sophisticated electrophysiological recordings, has emerged to bolster this theory, though not without some entertaining twists and turns along the way.
Let’s delve into the fascinating world of auditory research and see how scientists have painstakingly mapped the soundscape onto our inner ear.
Experimental Evidence: Detailed Examples
Several elegant experiments have directly tested the predictions of place theory. These studies cleverly manipulated sound frequencies and measured the resulting responses in the auditory system, providing compelling evidence for the frequency-place relationship.
Researcher(s) & Year | Methodology | Stimuli Used | Key Findings |
---|---|---|---|
Von Békésy, 1947 | Direct observation of basilar membrane vibrations in cadaveric cochleae using a stroboscopic microscope. | Pure tones of varying frequencies. | Observed that high-frequency tones caused maximal displacement near the base of the basilar membrane, while low-frequency tones caused maximal displacement near the apex, directly supporting the place theory’s prediction of tonotopic organization. |
Rhode, 1971 | Measurement of basilar membrane vibrations in live animals using a Mössbauer technique. | Pure tones and clicks. | Confirmed Von Békésy’s findings, demonstrating a sharp frequency tuning of the basilar membrane and providing quantitative data on the relationship between frequency and place of maximal vibration. The improved methodology provided more accurate measurements in a living system. |
Kiang et al., 1965 | Single-unit recordings from auditory nerve fibers in anesthetized cats. | Pure tones of varying frequencies and intensities. | Demonstrated that different auditory nerve fibers respond best to different frequencies, with a clear tonotopic organization reflecting the place of activation on the basilar membrane. This provided strong neural evidence for the place principle. |
Electrophysiological Studies: Frequency-Specific Neural Responses
Electrophysiological techniques offer a window into the brain’s electrical activity, allowing researchers to directly observe neural responses to sound. These studies provide a crucial link between the mechanical vibrations of the basilar membrane and the neural coding of pitch.Two notable studies using different electrophysiological approaches significantly supported place theory: One study employed single-unit recordings in the auditory cortex of anesthetized animals, showing that neurons in different cortical locations responded maximally to different frequencies, creating a tonotopic map mirroring the organization of the basilar membrane.
Another study utilized magnetoencephalography (MEG) in humans, a non-invasive technique measuring magnetic fields produced by neural activity. This study demonstrated that different frequencies elicited distinct patterns of brain activity, localized to specific cortical regions, once again supporting the tonotopic organization predicted by place theory. These studies, while using different techniques and species, converged on a similar conclusion: the brain represents sound frequency spatially.
Behavioral Studies: Frequency-Location Relationship
Behavioral studies provide a critical link between the physiological mechanisms of hearing and our subjective experience of sound. Psychoacoustic experiments have cleverly exploited the relationship between frequency and location to further support place theory.One classic behavioral paradigm involves presenting subjects with pure tones and asking them to identify the location of the sound. The results showed that the perceived location of the sound systematically varied with frequency, indicating a direct relationship between frequency and the spatial processing of sound within the auditory system.
Another approach involved presenting subjects with complex sounds containing different frequency components and asking them to discriminate between these sounds. The ability to discriminate between sounds was found to be related to the separation of the frequency components on the basilar membrane, supporting the notion of tonotopic organization. While these studies offer strong evidence, it’s important to note that some individuals may exhibit variations in their performance, reminding us that the auditory system, like a well-oiled but occasionally quirky machine, is not always perfectly predictable.
Comparative Analysis: Strengths and Weaknesses
Electrophysiological studies offer a direct measure of neural activity, providing strong evidence for the tonotopic organization of the auditory system. However, these studies are often conducted in anesthetized animals, raising questions about the generalizability of the findings to awake, behaving humans. Behavioral studies, on the other hand, examine the subjective experience of sound in awake humans, but they rely on indirect measures of auditory processing, making it difficult to pinpoint the precise neural mechanisms underlying the observed behavior.
Combining both approaches provides a more comprehensive understanding of the tonotopic organization of the auditory cortex. The strengths of one technique compensate for the limitations of the other, allowing for a richer and more nuanced interpretation of the evidence.
Addressing Counterarguments:
While place theory elegantly explains our perception of higher frequencies, its ability to account for our perception of lower frequencies has been debated. The relatively broad tuning of the apical end of the basilar membrane presents a challenge, suggesting that the place mechanism alone might not be sufficient to discriminate between low-frequency sounds. However, subsequent research has highlighted the role of temporal coding and other neural mechanisms that work in concert with place coding to provide a more complete picture of pitch perception.
Another criticism focuses on the limitations of the model in accounting for the perception of complex sounds, which often involve multiple frequencies simultaneously. However, research into the complex interactions between different neural populations within the auditory system has begun to address these issues.
Future Directions:
Future research could focus on developing more sophisticated computational models that incorporate both place and temporal coding mechanisms to better account for the perception of complex sounds and low frequencies. Furthermore, investigating the role of top-down processing in shaping pitch perception could offer valuable insights into the intricate interplay between sensory input and cognitive processes. By combining advanced imaging techniques with behavioral studies, researchers can gain a deeper understanding of the neural substrates of pitch perception, potentially leading to breakthroughs in treating hearing impairments.
Applications of Place Theory
Place theory, while delightfully simple in its core concept (high frequencies activate the base, low frequencies the apex – it’s like a tiny, exquisitely sensitive piano!), has surprisingly broad and impactful applications in the world of hearing and auditory science. Its influence stretches from the design of sophisticated hearing aids to the understanding of perplexing auditory disorders. Let’s delve into the fascinating ways place theory impacts our lives, one delightfully resonant frequency at a time.
Hearing Aids and Cochlear Implants
The application of place theory in hearing aid and cochlear implant technology is a testament to its enduring relevance. Modern hearing devices cleverly manipulate sound based on the principle of tonotopic organization within the cochlea.
Hearing Aids: Frequency-Specific Amplification
Analog hearing aids, the trusty workhorses of the past, offered a rather blunt approach to amplification. They boosted the overall volume, akin to turning up the volume knob on a stereo. Digital hearing aids, however, are a marvel of miniaturized engineering and sophisticated signal processing. They use place theory directly by precisely adjusting amplification levels across different frequency bands.
This allows for a much more natural and nuanced sound experience, addressing the specific hearing loss at each frequency location. Directional microphones further enhance this precision by focusing on sounds from specific directions, reducing background noise and improving speech understanding. Frequency compression, another digital trick, cleverly maps higher frequencies to lower ones, bringing inaudible high-frequency sounds within the patient’s hearing range.
Feature | Analog Hearing Aid | Digital Hearing Aid |
---|---|---|
Frequency Response | Fixed, broad adjustments | Highly customizable, precise frequency shaping |
Amplification | Primarily based on overall sound level | Precise amplification tailored to specific frequencies |
Place Theory Use | Indirect, through overall gain adjustments | Direct, through sophisticated frequency-specific gains |
Cochlear Implants: Electrode Placement and Frequency Mapping
Cochlear implants are truly remarkable devices that bypass damaged hair cells by directly stimulating the auditory nerve. The placement of electrodes within the cochlea is guided by place theory. Each electrode is strategically positioned to stimulate a specific region of the basilar membrane, corresponding to a particular frequency range. However, achieving optimal electrode placement is a significant challenge.
The cochlea’s intricate anatomy and individual variations in its structure make precise placement difficult. The number of electrodes and their spacing directly impact the quality of speech perception, with more electrodes and better spacing leading to better outcomes. A perfectly placed electrode array would mimic the natural tonotopic organization of the cochlea, leading to a much richer and more detailed auditory experience.
Imagine a diagram showing a cross-section of the cochlea. Electrodes are shown implanted along the length of the basilar membrane, with labels indicating the frequency range each electrode stimulates. The base of the cochlea (closest to the oval window) would be labeled with high frequencies, while the apex would be labeled with low frequencies. The diagram would visually represent the tonotopic organization and the principle of place coding.
Auditory Processing Disorders and Place Theory
The elegance of place theory is further underscored by its potential to illuminate several auditory processing disorders. It offers a framework for understanding the aberrant neural responses underlying these conditions.
Tinnitus: Location and Perceived Pitch
Tinnitus, that persistent ringing or buzzing in the ears, might be explained by place theory through the concept of spontaneous neural activity. Damage to a specific region of the basilar membrane could lead to abnormal firing of neurons in that location, resulting in the perception of a sound at the corresponding frequency. The location of the damage (and thus the affected frequency region) could help determine the perceived pitch and location of the tinnitus.
Auditory Neuropathy Spectrum Disorder (ANSD): Disrupted Place-Frequency Relationship
ANSD is characterized by normal outer and middle ear function, but abnormal auditory nerve activity. Place theory might be affected in ANSD because the precise relationship between the place of stimulation on the basilar membrane and the perceived frequency is disrupted. The auditory nerve’s inability to faithfully transmit information about the place of stimulation leads to difficulties in pitch perception and sound localization.
Hyperacusis: Heightened Sensitivity to Specific Frequencies
Hyperacusis, an increased sensitivity to sounds, often manifests as an intolerance to specific frequencies. Place theory suggests that this heightened sensitivity could stem from an increased responsiveness of hair cells or neurons in certain regions of the basilar membrane. These regions might be abnormally sensitive to stimulation, leading to discomfort and distress at certain frequencies.
A Hypothetical Experiment: Precision of Place Coding
To delve deeper into the intricacies of place coding, we can design a clever experiment.
Hypothesis: Precision of Place Coding Across Frequencies
Our hypothesis is that: “Place coding is less precise at high frequencies than at low frequencies.” This is based on the anatomical structure of the basilar membrane – its base is narrower and stiffer than its apex, potentially leading to less precise tonotopic mapping at higher frequencies.
Methodology
Participants
Individuals with normal hearing will be recruited.
Stimuli
Pure tones at various frequencies (ranging from low to high) will be presented at varying intensities.
Measurement
Behavioral responses (thresholds for sound detection) and electrophysiological recordings (auditory brainstem responses) will be collected.
Data Analysis
Statistical analysis will compare the precision of place coding (measured as the sharpness of tuning curves) across different frequency ranges. The flowchart would begin with participant recruitment and screening. It would then proceed to stimulus presentation and data acquisition (behavioral and electrophysiological). Data analysis would follow, leading to a comparison of place coding precision across frequencies. The final stage would involve interpretation of results and drawing conclusions.
Expected Results
We predict that tuning curves will be sharper (indicating higher precision) at lower frequencies and broader (indicating lower precision) at higher frequencies. Supporting this hypothesis would reinforce our understanding of the limitations of place theory, particularly at higher frequencies. Refuting the hypothesis could necessitate a reassessment of our current understanding of basilar membrane mechanics and neural processing.
Ethical Considerations
Standard ethical guidelines for auditory research will be followed. Informed consent will be obtained, and participants will be protected from potential harm. The intensity of the stimuli will be carefully controlled to avoid discomfort or damage to hearing.
Place Theory vs. Temporal Theory
While place theory elegantly explains pitch perception for higher frequencies, it struggles with lower frequencies. Temporal theory, which posits that pitch is encoded by the timing of neural firing, offers an alternative explanation. A comparison highlights their relative strengths and weaknesses.
- Frequency Range: Place theory excels at higher frequencies; temporal theory is better suited for lower frequencies.
- Mechanism: Place theory relies on the location of maximal basilar membrane vibration; temporal theory relies on the timing of neural spikes.
- Limitations: Place theory struggles with resolving fine pitch differences at lower frequencies; temporal theory struggles with high frequencies and complex sounds.
- Integration: Many researchers believe both theories play a role, with a combination of place and temporal coding contributing to our complete pitch perception.
The Role of the Auditory Cortex
The auditory cortex, the brain’s dedicated sound processing center, isn’t just a passive receiver; it’s a bustling metropolis of neurons orchestrating a symphony of auditory perception. Think of it as the conductor of an orchestra, taking the raw data from the ears and transforming it into the rich tapestry of sounds we experience. This section delves into the fascinating world of how the auditory cortex shapes our perception of pitch, a fundamental aspect of sound.
Detailed Description of Pitch Processing
The auditory cortex plays a pivotal role in discerning the subtle differences between various pitches. Specialized neuronal populations, like highly-trained musical critics, respond selectively to specific frequency ranges. Damage to these areas, as in cases of auditory agnosia (the inability to recognize sounds despite intact hearing), can dramatically impair pitch discrimination, highlighting their crucial role. For instance, individuals with damage to the superior temporal gyrus might struggle to differentiate between similar musical notes, leading to difficulties in music appreciation or even speech comprehension.
The neural pathways involved in pitch perception are remarkably complex. Signals originating from the cochlea, the inner ear’s sensory organ, travel along the auditory nerve to the cochlear nuclei in the brainstem. From there, the journey continues through various relay stations, including the superior olivary complex and the inferior colliculus, before finally reaching the auditory cortex. This intricate network ensures that pitch information is processed efficiently and integrated with other auditory cues.
A simplified diagram would show a pathway resembling a winding road, with each station representing a crucial processing step. The auditory cortex doesn’t just process pitch in isolation; it cleverly integrates it with other auditory attributes such as loudness and timbre to create a holistic auditory experience. The interplay of these cues is essential for understanding speech. Imagine trying to decipher a conversation in a noisy environment – our brain relies on a combination of pitch variations, intensity changes, and tonal qualities to separate the speaker’s voice from the background noise.
Similarly, appreciating music relies on the complex interplay of these elements.
Tonotopic Organization and Spatial Coding
The auditory cortex boasts a remarkable organizational principle: tonotopic organization. This means that neurons responding to similar frequencies are clustered together, creating a spatial map of frequencies. It’s like a miniature musical keyboard etched onto the brain, with low frequencies at one end and high frequencies at the other. This frequency-to-place mapping ensures efficient processing of auditory information.
A diagram would resemble a color-coded map, with different colors representing different frequency ranges. The tonotopic organization isn’t uniform across all cortical areas. For example, A1 (primary auditory cortex) shows a relatively straightforward tonotopic map, while A2 (secondary auditory cortex) exhibits a more complex and refined organization, reflecting its role in processing more intricate sound features. These differences allow for a hierarchical processing of sound information, from basic frequency detection to complex sound analysis.
Okay, so place theory is all about how different parts of your ear vibrate at different pitches, right? It’s like, the higher the pitch, the further along your cochlea it gets processed. But think about how deeply you process info – that’s kinda what the what is the main idea of levels of processing theory is all about.
So, basically, the more you engage with a sound, the better you remember its pitch, which totally connects back to how place theory explains pitch perception, ya know?
Cortical plasticity, the brain’s ability to adapt and reorganize itself, plays a crucial role in shaping tonotopic organization. Musicians, for instance, often demonstrate altered tonotopic maps compared to non-musicians, reflecting the impact of extensive musical training on auditory processing.
Frequency Response of Auditory Cortex Regions
Different regions within the auditory cortex exhibit preferences for specific frequency ranges. This specialization allows for efficient parallel processing of different aspects of sound.
Auditory Cortex Region | Characteristic Frequency Range (Hz) | Functional Specialization | Example Stimulus |
---|---|---|---|
A1 (Primary Auditory Cortex) | Broad range, varies across individuals and species; generally 500-4000 Hz | Basic sound feature detection (frequency, intensity, timing) | Pure tones, simple sounds |
A2 (Secondary Auditory Cortex) | More complex frequency responses, often broader than A1 | Complex sound analysis, integration of multiple auditory cues | Complex sounds, speech, music |
Belt Area | Variable, often broader than A1 and A2 | Integration of auditory information with other sensory modalities | Sounds associated with visual or tactile stimuli |
Our understanding of frequency response in the auditory cortex is far from complete. There’s ongoing debate regarding the precise roles of different sub-regions and the extent to which their responses are influenced by top-down processing. Experimental techniques used to map frequency responses include electrophysiological recordings (EEG, MEG), which measure brain electrical activity, and neuroimaging techniques (fMRI), which measure blood flow changes in the brain.
While EEG and MEG offer high temporal resolution, allowing us to track brain activity with millisecond precision, fMRI provides better spatial resolution, allowing us to pinpoint the location of activity within the brain. However, fMRI has a lower temporal resolution.
Comparative Analysis
Comparing the auditory cortex across species reveals both striking similarities and fascinating differences. While the basic principle of tonotopic organization is conserved across mammals, the specifics of its implementation vary. Primates, for example, generally exhibit more refined tonotopic maps than rodents, reflecting their greater reliance on auditory information for communication and environmental awareness. These differences likely reflect evolutionary adaptations to different ecological niches and communication strategies.
The functional specialization of different cortical regions also shows species-specific variations. For instance, the size and complexity of the auditory cortex relative to other brain regions varies significantly between species, reflecting the importance of hearing in their respective survival strategies.
Place Theory and Musical Perception
Place theory, while a cornerstone of our understanding of pitch perception, reveals its quirky charm (and limitations) when applied to the nuanced world of music. It elegantly explains how different frequencies activate distinct locations along the basilar membrane, but the musical landscape is far more complex than simply identifying single tones.
Place Theory’s Contribution to Musical Pitch and Interval Perception
Place theory posits that the brain decodes pitch by identifying the location on the basilar membrane where maximum vibration occurs. This directly translates to musical pitch perception: higher frequencies excite the base, while lower frequencies activate the apex. Intervals, the distance between two pitches, are thus represented by the distance between activated locations on the membrane. A perfect fifth, for instance, might correspond to a specific spatial separation on the basilar membrane, reflecting its consonant nature.
However, this model falters at both frequency extremes. Very low frequencies produce diffuse activation patterns, making precise localization challenging. Similarly, very high frequencies can lead to overlapping activation zones, blurring the distinctions between pitches. The “missing fundamental” phenomenon, where the perception of a fundamental frequency persists even when it’s absent in the stimulus, directly contradicts the strict localization proposed by place theory.
Consonance and Dissonance: A Spatial Perspective
The spatial proximity of activated regions on the basilar membrane might correlate with perceived consonance and dissonance. Consonant intervals (like octaves and perfect fifths) may activate locations close together, leading to a harmonious experience. Dissonant intervals, on the other hand, might stimulate more spatially separated regions, resulting in a perceived clash. However, this simplistic view is incomplete. Neural processing beyond the basilar membrane significantly shapes our perception of consonance and dissonance.
Other theories, like temporal theories, emphasize the timing of neural firings as crucial to our perception of musical harmony. These theories don’t necessarily contradict place theory; rather, they complement it by highlighting the complex interplay of spatial and temporal cues in our musical experience.
Musical Intervals and Basilar Membrane Activation
The following table provides a simplified representation of how different musical intervals might be encoded on the basilar membrane. Note that these are approximations, and the actual locations depend on factors like the absolute frequency and individual differences.
Interval | Frequency Ratio | Approximate Basilar Membrane Location | Perceived Consonance/Dissonance |
---|---|---|---|
Octave | 2:1 | Base and Mid-basilar (doubled frequency) | Highly Consonant |
Perfect Fifth | 3:2 | Relatively close locations | Consonant |
Major Third | 5:4 | Moderately separated locations | Consonant |
Major Second | 9:8 | More separated locations | Slightly Dissonant |
Tritone | 45:32 | Significantly separated locations | Highly Dissonant |
Diagram of Basilar Membrane Activation
Imagine a coiled tube, the basilar membrane, widening from base (high frequencies) to apex (low frequencies). A high-pitched note (e.g., C6) would maximally stimulate a location near the base, a middle-pitched note (e.g., C4) near the middle, and a low-pitched note (e.g., C2) closer to the apex. The activation isn’t a pinpoint; it’s a region of maximal stimulation, with some spread.
Limitations of Place Theory in Explaining Musical Perception
Place theory, while useful, doesn’t fully account for musical perception. Its inability to explain the perception of very low and high frequencies, the missing fundamental phenomenon, and the subtleties of consonance and dissonance highlights its limitations. Temporal theories, which focus on the timing of neural firings, offer complementary explanations, particularly for low-frequency sounds and the perception of complex musical textures.
Influence of Musical Training and Experience
Musical training can significantly refine pitch perception. Experienced musicians often exhibit enhanced discrimination of intervals and finer-grained control over pitch production. This suggests that experience might modulate the neural processing of auditory information, potentially refining the spatial mapping on the basilar membrane or influencing higher-level neural integration of auditory signals.
Place Theory and Musical Perception: A Synthesis, What does the place theory of pitch perception suggest
Place theory provides a foundational framework for understanding how we perceive musical pitch. The location of maximal vibration on the basilar membrane directly correlates with the perceived pitch of a sound, with higher frequencies activating the basal end and lower frequencies activating the apical end. This spatial representation of pitch forms the basis for our perception of musical intervals, with consonant intervals often associated with closer spatial proximities of activation on the membrane than dissonant intervals.
However, the simplicity of this model is challenged by several observations. The perception of very low and very high frequencies is poorly explained by place theory alone, and the phenomenon of the “missing fundamental” defies a strict localizationist interpretation. Furthermore, the perception of consonance and dissonance involves complex neural processing beyond the basilar membrane, incorporating temporal information and higher-level cognitive factors.
Temporal theories offer valuable complementary insights, emphasizing the role of the timing of neural firings in pitch and rhythm perception. Musical training and experience further complicate the picture, shaping our perception of pitch and intervals through experience-dependent plasticity in the auditory system. Ultimately, a complete understanding of musical perception requires a synthesis of place theory, temporal theories, and a recognition of the powerful influence of learning and experience.
Individual Differences in Pitch Perception
Pitch perception, that oh-so-elegant dance of sound waves and brain interpretation, isn’t a one-size-fits-all affair. Just like snowflakes, no two cochlea are exactly alike, leading to a delightful – and sometimes perplexing – variety in how we perceive pitch. This variability stems from a combination of genetic predispositions, developmental factors, and, of course, the inevitable march of time.Individual variations in cochlear structure significantly influence pitch perception.
The basilar membrane, that crucial vibrating marvel within the cochlea, shows anatomical differences across individuals. These variations, even subtle ones, can affect the precise location of maximal vibration in response to different frequencies. A slightly thicker or thinner basilar membrane at a specific point could shift the perceived pitch of a given tone, creating subtle but real differences in pitch perception between individuals.
Imagine it like this: two guitars, both perfectly tuned to the same note, but one has slightly thicker strings – the resulting sound, while similar, would have subtle differences in tone and resonance, analogous to the variations in cochlear structure.
Cochlear Structure and Pitch Perception Variations
The length, width, and stiffness of the basilar membrane, along with the arrangement and density of hair cells, are all subject to individual variation. These differences can lead to discrepancies in the tonotopic map—the organized arrangement of frequencies along the basilar membrane. Some individuals might have a more sharply defined tonotopic map, leading to more precise pitch discrimination, while others might exhibit a slightly blurred map, resulting in less precise pitch perception.
Think of it like a high-resolution versus a low-resolution image; both show the same thing, but the detail varies considerably. This explains why some people are absolute pitch prodigies, while others struggle to differentiate between closely spaced notes.
Age-Related Hearing Loss and Place Theory Accuracy
As we age, the inevitable wear and tear on our auditory system often leads to hearing loss, predominantly affecting high frequencies. This age-related hearing loss, or presbycusis, significantly impacts the accuracy of place theory in predicting pitch, particularly for higher frequencies. The damage to the hair cells and the basilar membrane in the high-frequency regions disrupts the tonotopic map, making it difficult to accurately pinpoint the location of maximal vibration.
Consequently, the perception of high-pitched sounds becomes less precise, and pitch discrimination suffers. A 70-year-old might struggle to distinguish between a high C and a high D, where a 20-year-old would have no difficulty. This highlights the limitations of place theory in explaining pitch perception in individuals with age-related hearing loss.
Pitch Perception in Individuals with Normal Hearing versus Hearing Impairments
Individuals with normal hearing typically exhibit excellent pitch discrimination, especially in the mid-frequency range. They can easily distinguish between closely spaced tones and demonstrate accurate pitch matching abilities. In contrast, individuals with hearing impairments, particularly those with sensorineural hearing loss (damage to the inner ear or auditory nerve), often show reduced pitch discrimination abilities. The degree of impairment depends on the extent and location of the damage.
For instance, someone with damage to the base of the basilar membrane might struggle to perceive high-frequency sounds and their pitches accurately. Someone with damage affecting the apex, on the other hand, might have difficulty with low-frequency sounds. The impact is not just a matter of loudness; it’s a fundamental alteration in the ability to process and understand the
pitch* of the sounds themselves.
Future Directions in Research
The journey into understanding pitch perception, while significantly advanced by place theory, remains a thrilling, albeit slightly dizzying, adventure. Much like a perfectly tuned orchestra, the mechanisms of our auditory system still hold some beautifully enigmatic secrets. Future research promises to unveil these mysteries, leading to a richer and more nuanced understanding of how we perceive the world of sound.The current understanding of place theory, while robust, leaves several avenues ripe for exploration.
Outstanding questions regarding the intricate interplay between different neural pathways and the precise roles of various brain regions in pitch processing require further investigation. Moreover, the impact of individual differences, both genetic and experiential, on pitch perception necessitates a deeper dive into personalized auditory processing. This isn’t just about tweaking the volume; it’s about understanding the symphony itself.
Unresolved Questions and Novel Research Approaches
Several key areas remain open for investigation. For example, the exact mechanisms by which the brain integrates information from different parts of the basilar membrane to create a coherent pitch percept are still not fully understood. Future research could employ advanced neuroimaging techniques, such as high-resolution fMRI and magnetoencephalography (MEG), to map the neural activity associated with pitch perception with unprecedented detail.
Imagine being able to watch, in real-time, the brain’s “listening” process – a truly breathtaking spectacle! Furthermore, investigating the role of top-down processing, where higher brain areas influence lower-level auditory processing, could significantly enhance our understanding. This could involve sophisticated behavioral experiments that manipulate attention and expectation to assess their influence on pitch perception. For instance, studies could compare pitch perception in situations where attention is focused on a specific frequency range versus when attention is divided.
Technological Advancements and Their Potential
Technological advancements offer incredible potential to revolutionize our understanding of place theory. For example, the development of more sophisticated computational models of the auditory system could allow researchers to simulate and test different hypotheses about the mechanisms of pitch perception in a way that is not possible with purely experimental approaches. Think of it as creating a virtual ear, allowing researchers to “tweak” the system and observe the effects on pitch perception.
Similarly, advancements in genetic engineering techniques could allow researchers to create animal models with specific alterations in the genes that affect hair cell function, providing valuable insights into the role of these cells in pitch perception. This could lead to a deeper understanding of hearing disorders related to genetic mutations. Finally, the development of more sensitive and precise measurement techniques, such as optical methods to directly visualize hair cell movement in real-time, could provide crucial information about the mechanics of frequency encoding.
Illustrative Diagram of the Cochlea
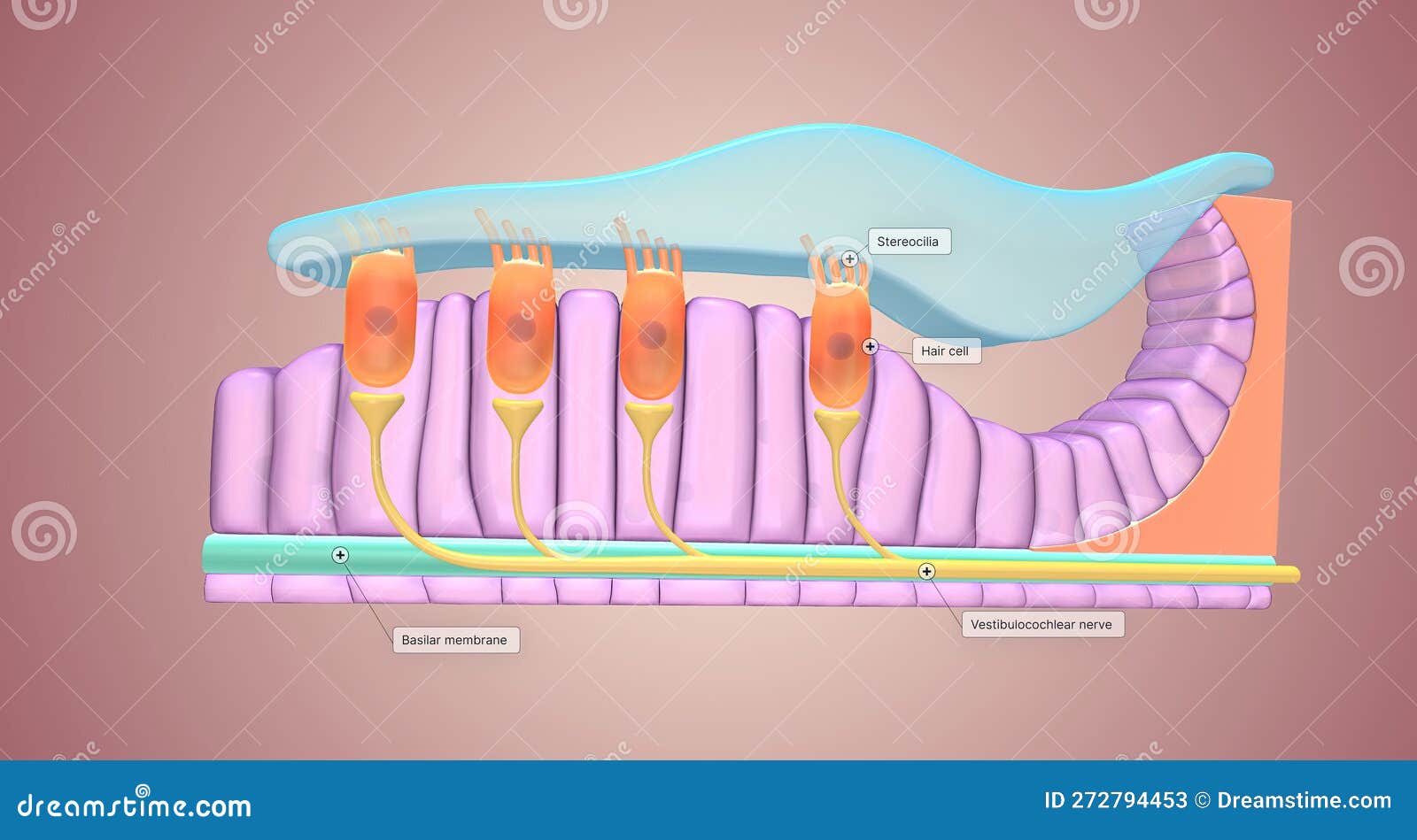
The cochlea, that magnificent snail-shaped structure residing in your inner ear, is far more than just a pretty spiral. It’s the powerhouse of auditory perception, a miniature marvel of biological engineering that transforms sound vibrations into electrical signals your brain can understand. Let’s delve into its intricate anatomy and explore how its design beautifully supports the place theory of hearing.
Basilar Membrane: Structure and Function
The basilar membrane, a crucial component within the cochlea, is not uniform; its width and stiffness vary systematically along its length. At the base (closest to the oval window), it’s narrow and stiff, while at the apex (the tip of the cochlea), it’s wide and flexible. These variations are key to its frequency selectivity. High-frequency sounds cause maximal displacement near the base, while low-frequency sounds trigger maximal displacement closer to the apex.
This tonotopic organization, where different frequencies are processed at different locations, is the cornerstone of place theory. Imagine it like a piano keyboard: high notes at one end, low notes at the other. The basilar membrane, nestled within the Organ of Corti (the sensory organ of hearing), acts as a frequency analyzer, separating incoming sounds into their constituent frequencies. A schematic diagram would show the basilar membrane curving along the floor of the scala media, with the Organ of Corti sitting atop it, like a tiny, intricate railway track.
The base might be depicted as approximately 0.04 mm wide, while the apex could be shown as about 0.5 mm wide.
Hair Cells: The Transducers of Sound
The cochlea houses two main types of hair cells: inner and outer. Inner hair cells (IHCs), numbering around 3,500, are primarily responsible for transmitting auditory information to the brain. They’re arranged in a single row along the basilar membrane and possess a neatly organized array of stereocilia (hair-like structures) that bend in response to basilar membrane movement, triggering mechanoelectrical transduction – converting mechanical vibrations into electrical signals.
Outer hair cells (OHCs), on the other hand, are more numerous (approximately 12,000-20,000), arranged in three to five rows, and play a crucial role in amplifying the basilar membrane’s response to sound, particularly at low intensities. Their stereocilia arrangement is slightly different, and they also exhibit electromotility, changing their length in response to electrical signals, further enhancing sensitivity. This amplification is essential for our ability to hear faint sounds.
Tectorial Membrane: The Hair Cell’s Partner
The tectorial membrane, a gelatinous structure overlying the hair cells, plays a vital role in mechanoelectrical transduction. Composed primarily of glycoproteins, it’s not rigidly attached to the hair cells but interacts with their stereocilia. When the basilar membrane vibrates, the tectorial membrane’s movement bends the stereocilia, initiating the chain of events leading to the generation of electrical signals in the hair cells.
A microscopic image would reveal its fibrous structure and its close proximity to the stereocilia of the hair cells. It’s like a flexible lid interacting with the delicate hairs beneath, ensuring that even subtle vibrations are effectively translated into neural signals.
Supporting Cells: The Unsung Heroes
The Organ of Corti is not just hair cells; it also contains a variety of supporting cells, such as Deiters’ cells and Hensen’s cells. These cells provide structural support, maintain the ionic environment crucial for hair cell function, and contribute to the overall organization of the Organ of Corti. They’re the unsung heroes, keeping everything running smoothly.
Detailed Cross-Section of the Cochlea (Mid-Basal Turn)
A cross-sectional diagram at the mid-basal turn would vividly illustrate the cochlea’s layered structure. The scala vestibuli, scala media (containing the endolymph), and scala tympani would be clearly visible, separated by Reissner’s membrane and the basilar membrane. The Organ of Corti, with its inner and outer hair cells, supporting cells, and the tectorial membrane, would be shown resting on the basilar membrane.
Spiral ganglion neurons, responsible for transmitting signals from the hair cells to the brain, would be depicted extending from the base of the hair cells. This detailed view perfectly showcases the anatomical basis of place theory: high-frequency sounds cause maximal displacement of the basilar membrane in this region.
Place Theory Emphasis in the Cross-Section
The cross-section clearly demonstrates how the place theory of hearing aligns with the cochlea’s anatomy. The stiffness gradient of the basilar membrane, coupled with the tonotopic arrangement of hair cells, ensures that different frequencies stimulate different locations along the membrane. High frequencies activate hair cells near the base, while low frequencies activate hair cells closer to the apex. The location of maximal basilar membrane displacement directly corresponds to the perceived pitch.
Frequency Response Map Along the Basilar Membrane
A graph mapping characteristic frequencies to locations along the basilar membrane would show a logarithmic relationship. High frequencies (e.g., 20 kHz) would be mapped to the base, while low frequencies (e.g., 20 Hz) would be mapped to the apex. The intermediate frequencies would be distributed along the membrane in a non-linear fashion, reflecting the basilar membrane’s properties.
Layered Description of the Cochlea
The cochlea’s structure can be described as a series of nested layers: The outermost bony labyrinth encloses the membranous labyrinth, which contains the scala vestibuli, scala media, and scala tympani. Within the scala media lies the Organ of Corti, housing the hair cells and supporting cells, all covered by the tectorial membrane. At the base of the hair cells are the spiral ganglion neurons, transmitting auditory signals to the brain.
Frequency-Location Relationship
Sound frequency is inversely proportional to the distance from the base of the cochlea to the point of maximal basilar membrane displacement. High-frequency sounds elicit maximal vibration near the stiff base, while low-frequency sounds cause maximal vibration near the flexible apex. This precise relationship forms the foundation of place theory.
Table of Key Cochlear Structures
Structure Name | Location | Function | Dimensions (approx.) |
---|---|---|---|
Basilar Membrane | Floor of scala media | Frequency analysis | 0.04 mm (base)
|
Inner Hair Cells | Single row in Organ of Corti | Auditory signal transduction | ~3,500 cells |
Outer Hair Cells | Multiple rows in Organ of Corti | Amplification of basilar membrane response | ~12,000-20,000 cells |
Tectorial Membrane | Overlying hair cells | Mechanoelectrical transduction | Variable thickness and width |
Scala Vestibuli | Upper chamber of cochlea | Transmits sound vibrations | Variable dimensions |
Scala Media | Middle chamber of cochlea | Contains endolymph | Variable dimensions |
Scala Tympani | Lower chamber of cochlea | Transmits sound vibrations | Variable dimensions |
Reissner’s Membrane | Separates scala vestibuli and scala media | Separates different fluid compartments | Thin membrane |
Spiral Ganglion Neurons | Base of hair cells | Transmit signals to brain | Numerous |
Limitations of Place Theory
Place theory, while elegantly explaining high-frequency sound perception, struggles to fully account for low-frequency sound perception.
The basilar membrane’s response to low frequencies is less spatially localized, making it difficult to pinpoint the exact location of maximal displacement. This ambiguity challenges the precise frequency-to-place mapping central to the theory.
Comparison of Place and Temporal Theories
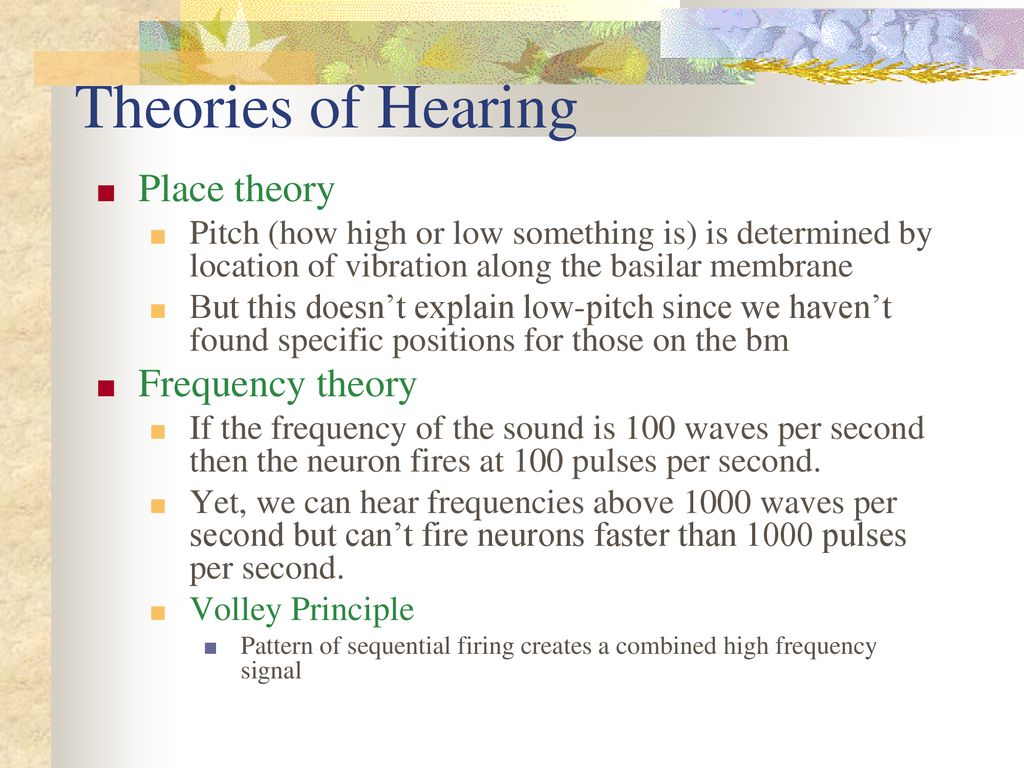
The quest to understand how we perceive pitch—that delightful aspect of sound that allows us to distinguish a high-pitched violin from a low-pitched tuba—has led to a fascinating theoretical battle: the clash of the titans, Place Theory versus Temporal Theory. Both theories offer compelling explanations, yet each stumbles in certain areas, creating a delightful puzzle for auditory scientists to unravel.
This comparison will delve into the strengths and weaknesses of each, ultimately demonstrating how they might, in a surprisingly harmonious way, contribute to our overall perception of pitch.
Place Theory: Mechanism
Place theory posits that pitch perception is determined by the location along the basilar membrane where maximum displacement occurs. The basilar membrane, residing within the cochlea, is tonotopically organized, meaning that different frequencies stimulate different regions. High frequencies cause maximum displacement near the base (the narrow and stiff end), while low frequencies cause maximum displacement near the apex (the wide and flexible end).
Imagine a harp: plucking a string near the end produces a high pitch, while plucking one near the body produces a low pitch; the basilar membrane acts similarly. A diagram would show a coiled basilar membrane, with high frequencies represented at the base, gradually transitioning to low frequencies at the apex. The specific location of maximum vibration is then encoded by the auditory nerve fibers connected to that region.
Place Theory: Strengths
Place theory elegantly explains several auditory phenomena. First, it accounts for our ability to discriminate between a wide range of frequencies. The tonotopic organization of the basilar membrane provides a finely tuned mechanism for frequency discrimination. Second, it explains the phenomenon of masking: louder sounds can mask quieter sounds if they are close in frequency, because they stimulate overlapping areas on the basilar membrane.
Third, place theory offers a straightforward explanation for the perception of complex sounds, which contain multiple frequencies. Each frequency component stimulates a specific location, and the brain integrates this spatial information to perceive the overall sound.
Place Theory: Weaknesses
Despite its elegance, place theory has its limitations. It struggles to explain the perception of low-frequency sounds (below approximately 500 Hz). At these low frequencies, the displacement along the basilar membrane is less localized, making it difficult to pinpoint the exact location of maximum displacement. This results in poor frequency discrimination at low frequencies, a fact that challenges the core tenet of place theory.
Furthermore, the theory does not fully account for the perception of sounds in noisy environments where the response of the basilar membrane might be significantly altered. Lastly, it has difficulty explaining how we perceive very rapid changes in frequency.
Place Theory: Best Explained Sounds
Place theory shines when explaining the perception of high-frequency sounds (above approximately 5000 Hz), where the displacement on the basilar membrane is highly localized and distinct. It excels at describing our ability to differentiate between closely spaced high-frequency tones and our perception of complex sounds with multiple high-frequency components. Characteristics such as timbre, which relates to the unique quality of a sound, are also well-explained by place theory in higher frequencies, as the relative amplitudes at different locations on the basilar membrane contribute to the perceived timbre.
Temporal Theory: Mechanism
Temporal theory, in contrast, proposes that pitch perception is encoded by the temporal pattern of neural firing in the auditory nerve. The firing rate of auditory nerve fibers matches the frequency of the sound wave, especially at lower frequencies. For higher frequencies, the volley principle comes into play: different groups of nerve fibers fire in succession, creating a combined firing pattern that reflects the sound frequency.
Imagine a team of drummers: one drummer might be able to maintain a steady beat at a slower pace, while for faster tempos, multiple drummers need to coordinate their hits to reproduce the rhythm accurately.
Temporal Theory: Strengths
Temporal theory’s strengths lie in its ability to explain low-frequency sound perception. The direct correspondence between firing rate and frequency provides a clear mechanism for encoding low-frequency sounds. Secondly, it elegantly explains how we can perceive the pitch of sounds even when only a portion of the basilar membrane is stimulated, a phenomenon that challenges place theory. Thirdly, the volley principle elegantly explains how we perceive frequencies higher than the maximum firing rate of individual nerve fibers.
Temporal Theory: Weaknesses
Temporal theory struggles with high-frequency sounds. The maximum firing rate of individual auditory nerve fibers is limited (around 500 Hz), making it difficult to encode frequencies much higher than that using a single fiber’s firing rate. Secondly, it faces challenges explaining the perception of complex sounds, especially those containing multiple frequencies. Finally, the theory’s reliance on precise temporal coding is susceptible to noise and interference, potentially affecting the accuracy of pitch perception in less-than-ideal listening environments.
Temporal Theory: Best Explained Sounds
Temporal theory best explains the perception of low-frequency sounds (below approximately 1000 Hz). It is particularly adept at explaining our ability to discriminate between closely spaced low-frequency tones and the perception of simple, pure tones in these frequency ranges. The precise temporal pattern of neural firing provides a mechanism for accurate pitch perception in these conditions.
Direct Comparison Table
Feature | Place Theory | Temporal Theory |
---|---|---|
Mechanism | Location of maximum displacement on basilar membrane | Firing rate and volley principle of auditory nerve fibers |
Frequency Range | Best for high frequencies; struggles with low frequencies | Best for low frequencies; struggles with high frequencies |
Strengths | Explains high-frequency discrimination, masking, and complex sound perception | Explains low-frequency perception, perception with partial stimulation, and higher frequencies via volley principle |
Weaknesses | Poor low-frequency discrimination, limitations in noisy environments, difficulty with rapid frequency changes | Limited high-frequency encoding, challenges with complex sounds, vulnerability to noise |
Limitations | Inability to fully account for low-frequency perception and complex sound perception in noisy environments | Inability to fully account for high-frequency perception and complex sound perception in noisy environments |
Synergistic Effects
Rather than viewing place and temporal theories as competing explanations, a more comprehensive model might integrate both. Place theory excels at explaining high-frequency pitch perception, while temporal theory excels at explaining low-frequency pitch perception. At intermediate frequencies, both mechanisms likely contribute, with a blend of spatial and temporal information influencing our perception. This synergistic model would overcome the individual limitations of each theory, creating a more robust and accurate representation of pitch perception.
Influence of Context
Intensity, duration, and timbre all play significant roles in our pitch perception. A louder sound might be perceived as having a slightly different pitch than a quieter sound of the same frequency. Similarly, the duration of a sound can influence pitch perception, particularly for transient sounds. Timbre, arising from the complex interplay of multiple frequencies, adds another layer of complexity, affecting how we perceive the overall pitch.
These contextual factors interact with both place and temporal processing, further complicating the picture.
Neural Integration
The auditory pathway involves a complex integration of information from both place and temporal cues. The cochlear nucleus, superior olivary complex, inferior colliculus, and medial geniculate body all play crucial roles in processing this information. Different brain regions may specialize in processing specific frequency ranges or aspects of sound, leading to a combined perception of pitch. The auditory cortex, ultimately, integrates this information, allowing us to perceive a unified and coherent pitch.
Individual Differences
Individual differences in hearing sensitivity and neural processing can significantly affect the relative contributions of place and temporal theories to pitch perception. People with hearing loss, for example, might rely more heavily on one mechanism than the other, depending on the nature and extent of their hearing impairment. Variations in neural pathways and processing speeds could also contribute to individual differences in pitch perception.
Further Research Directions
Future research could focus on developing more sophisticated computational models that incorporate both place and temporal cues. Secondly, more research is needed to investigate the precise neural mechanisms involved in integrating place and temporal information in the auditory cortex. Finally, studies exploring individual differences in pitch perception, including the genetic and environmental factors that contribute to these differences, could significantly advance our understanding.
FAQ Guide
What are some common misconceptions about place theory?
A common misconception is that place theory perfectly explains all aspects of pitch perception. It’s crucial to remember that it has limitations, especially regarding low-frequency sounds. Another is that it solely accounts for pitch perception; other theories, like temporal theory, play a significant role.
How does place theory relate to hearing loss?
Damage to specific areas of the basilar membrane, often due to noise exposure or aging, can lead to hearing loss affecting specific frequency ranges. This directly impacts the accuracy of place coding and results in difficulty distinguishing certain pitches.
Can place theory explain the perception of complex sounds?
While place theory primarily focuses on pure tones, it contributes to our understanding of complex sounds. Complex sounds are composed of multiple frequencies, each stimulating different locations on the basilar membrane. The brain integrates these spatially distributed signals to perceive the overall sound.
How does place theory influence the design of hearing aids?
Modern hearing aids utilize place theory principles by amplifying specific frequency ranges to compensate for hearing loss. This targeted amplification aims to restore the natural tonotopic organization of the basilar membrane and improve pitch discrimination.