Is string theory dead? Nah, fam, that’s a major oversimplification. String theory’s a wild ride—a super ambitious attempt to unify all the forces of nature, from the tiniest particles to the biggest cosmic events. It’s been around for decades, promising a beautiful, elegant explanation of everything, but experimental verification? That’s been the major roadblock.
This exploration dives deep into the current status of string theory, weighing its successes against the challenges it faces in the real world.
From its initial development and early promises to the current struggles with experimental verification, we’ll unpack the complexities. We’ll examine the energy scales needed to test its predictions, the technological hurdles, and the mathematical intricacies. We’ll also check out competing theories and explore the fascinating landscape of string theory solutions, touching on its influence in other areas of physics.
Get ready to level up your understanding!
Historical Context of String Theory
String theory’s emergence wasn’t a sudden revelation but a gradual evolution driven by persistent attempts to reconcile quantum mechanics with general relativity, a challenge that had baffled physicists for decades. Its roots lie in the study of the strong nuclear force, initially conceived as a way to describe the interactions of hadrons, particles composed of quarks. The early successes, though ultimately superseded, laid the groundwork for the more ambitious theory we know today.The initial development of string theory in the late 1960s and early 1970s saw it presented as a model for hadrons.
Instead of point-like particles, it proposed fundamental entities as one-dimensional vibrating strings. The different vibrational modes of these strings corresponded to different hadrons, offering a potentially elegant explanation for the particle zoo then being discovered in high-energy experiments. While this “hadronic string theory” ultimately failed to accurately predict experimental results, it provided the conceptual foundation for what would become a much broader and more ambitious theory.
Key Figures and Their Contributions
The evolution of string theory involved the contributions of numerous brilliant minds. Gabriele Veneziano’s discovery of a mathematical formula describing the scattering of hadrons, based on the Euler beta function, marked a crucial early step. This formula unexpectedly possessed properties consistent with both quantum mechanics and relativistic invariance. Leonard Susskind, Holger Bech Nielsen, and others subsequently interpreted this formula as describing the interactions of vibrating strings.
Subsequently, Yoichiro Nambu, T. Goto, and others showed that this string model could be derived from a relativistic action principle. The shift from a model of hadrons to a theory of quantum gravity came later, with the recognition that one of the vibrational modes corresponded to a massless spin-2 particle, consistent with the graviton, the hypothetical quantum of gravity.
This realization dramatically changed the trajectory of string theory, transforming it from a model of strong interactions to a potential theory of everything.
Initial Excitement and Expectations
The initial excitement surrounding string theory stemmed from its potential to unify all fundamental forces of nature within a single framework. It offered a path toward a quantum theory of gravity, a long-sought goal that had eluded physicists for decades. The elegance and mathematical sophistication of the theory, combined with its potential to solve some of the deepest mysteries of the universe, fueled immense optimism.
String theory promised to explain not only the fundamental particles and forces but also the origin and evolution of the universe, including its cosmological constants and the nature of spacetime itself. This ambition, however, also meant that verifying the theory experimentally would be incredibly challenging, requiring energies far beyond the reach of current technology. The expectation was that a deeper understanding of the theory itself, and the development of testable predictions, would eventually lead to experimental verification.
This expectation, while yet unfulfilled, remains a driving force behind ongoing research in the field.
Current Status of Experimental Verification
String theory, despite its elegant mathematical framework and potential to unify fundamental forces, remains stubbornly elusive to direct experimental verification. The challenge lies not in a lack of theoretical effort, but rather in the immense energy scales and subtle signatures involved, placing it far beyond the reach of current experimental capabilities. This section will delve into the current state of experimental evidence, the hurdles in designing testable experiments, and potential avenues for future breakthroughs.
Current Experimental Evidence and its Relevance to String Theory
The current state of experimental evidence offers neither robust support nor definitive refutation of string theory. The theory operates at energy scales far beyond the reach of current particle accelerators, meaning direct observation of its predicted phenomena remains impossible. Indirect tests, seeking to constrain the theory’s parameter space or identify potential signatures, have yielded limited results. The following table summarizes some key experimental efforts and their relevance:
Experiment | Result | Relevance to String Theory | Level of Support/Refutation |
---|---|---|---|
Large Hadron Collider (LHC) searches for supersymmetric particles | No conclusive evidence for supersymmetry found, though some models remain viable. | Many string theory models predict supersymmetry; lack of observation weakens (but doesn’t rule out) these models. | Moderate Refutation (for specific supersymmetric string models) |
Precision measurements of gravitational constants | Consistent with General Relativity within experimental uncertainties. | String theory modifications to gravity are generally subtle and difficult to detect at currently accessible energy scales. | Weak Refutation (no significant deviation from GR observed) |
Cosmic Microwave Background (CMB) observations | Highly consistent with the standard cosmological model (ΛCDM). | Some string-inspired models attempt to explain dark energy or dark matter, but no conclusive evidence points to these models over standard alternatives. | Weak Support (no unique string theory signature observed) |
Challenges in Designing Experiments to Test String Theory Predictions
Designing experiments to directly test string theory faces significant challenges across multiple domains:
The following points Artikel the major obstacles in experimentally verifying string theory predictions:
- Energy Scales: String theory predicts fundamental structures at the Planck scale (approximately 10 19 GeV), vastly exceeding the energy levels achievable in current particle accelerators (LHC’s maximum energy is around 13 TeV). Bridging this enormous gap requires a technological leap of unprecedented scale.
- Observable Signatures: While string theory predicts various phenomena like extra dimensions and exotic particles, these signatures are often highly suppressed at low energies or masked by background noise. Distinguishing these subtle effects from standard model processes or other competing theories presents a formidable challenge.
- Technological Limitations: Detecting the faint signals predicted by string theory requires advancements in detector technology capable of achieving unparalleled sensitivity and precision. Data analysis techniques need to be sophisticated enough to handle the massive datasets generated, and creating the extreme conditions (e.g., high energy densities) required to probe the Planck scale remains far beyond current capabilities.
Major Obstacles Preventing Experimental Verification
The obstacles to experimental verification can be categorized as follows:
Obstacle Category | Specific Obstacle | Explanation |
---|---|---|
Fundamental Obstacles | Existence of extra dimensions | The compactification of extra dimensions predicted by string theory may be at energy scales far beyond our reach, making their direct observation extremely difficult. |
Fundamental Obstacles | Landscape of possible vacua | The vast number of possible string theory vacua makes it difficult to pinpoint the specific vacuum representing our universe, hindering the ability to make precise, testable predictions. |
Technological Obstacles | Need for significantly higher energy accelerators | Achieving the energy scales required to probe string theory phenomena necessitates the construction of accelerators many orders of magnitude more powerful than the LHC, representing a monumental engineering challenge. |
Technological Obstacles | Development of new detection methods | Detecting the subtle signatures predicted by string theory requires innovative detection techniques with unprecedented sensitivity and resolution. |
Theoretical Obstacles | Lack of a complete non-perturbative formulation | The absence of a complete non-perturbative formulation of string theory limits our ability to make precise predictions for low-energy phenomena, hindering experimental testing. |
Theoretical Obstacles | Difficulty in making precise predictions | Even with a complete formulation, extracting precise, experimentally testable predictions from string theory remains a significant challenge. |
Comparison of Research Group Approaches to Experimental Verification
Various research groups employ diverse methodologies to indirectly test aspects of string theory. These approaches often focus on specific predictions or constraints, leading to a fragmented but active research landscape.
Research Group | Methodology | Target Prediction | Current Status |
---|---|---|---|
Example Group 1 (Hypothetical) | Precision cosmology measurements | Constraints on string-inspired dark energy models | Ongoing research, yielding some constraints but no definitive evidence. |
Example Group 2 (Hypothetical) | Analysis of LHC data for exotic particle signatures | Search for particles predicted by specific string theory models (e.g., Kaluza-Klein modes) | No conclusive evidence found to date. |
Example Group 3 (Hypothetical) | Development of novel string phenomenology models | Predicting observable signatures at lower energies | Active theoretical work, with some promising but unverified predictions. |
Novel Experimental Approaches for String Theory Verification
While direct verification remains a distant prospect, several novel approaches could potentially yield breakthroughs within the next two decades:
Approach | Principle | Challenges | Expected Outcomes |
---|---|---|---|
Advanced Gravitational Wave Detectors | Detect subtle gravitational wave signatures from string-scale processes. | Extremely faint signals, requiring significant improvements in detector sensitivity and noise reduction. | Potential detection of signals indicating extra dimensions or modifications to gravity predicted by string theory. |
Next-Generation Particle Accelerators | Achieve higher energy collisions to probe higher energy scales, potentially revealing string-scale phenomena. | Enormous engineering challenges and cost, requiring international collaborations. | Potential discovery of exotic particles or deviations from the standard model predicted by string theory. |
Precision Measurements of Fundamental Constants | Search for minute variations in fundamental constants over cosmological timescales, potentially indicating the presence of extra dimensions or other string-theoretic effects. | Requires extremely precise measurements and sophisticated data analysis techniques to account for systematic errors. | Potential observation of variations inconsistent with the standard model, hinting at string-theoretic effects. |
Mathematical Challenges and Developments
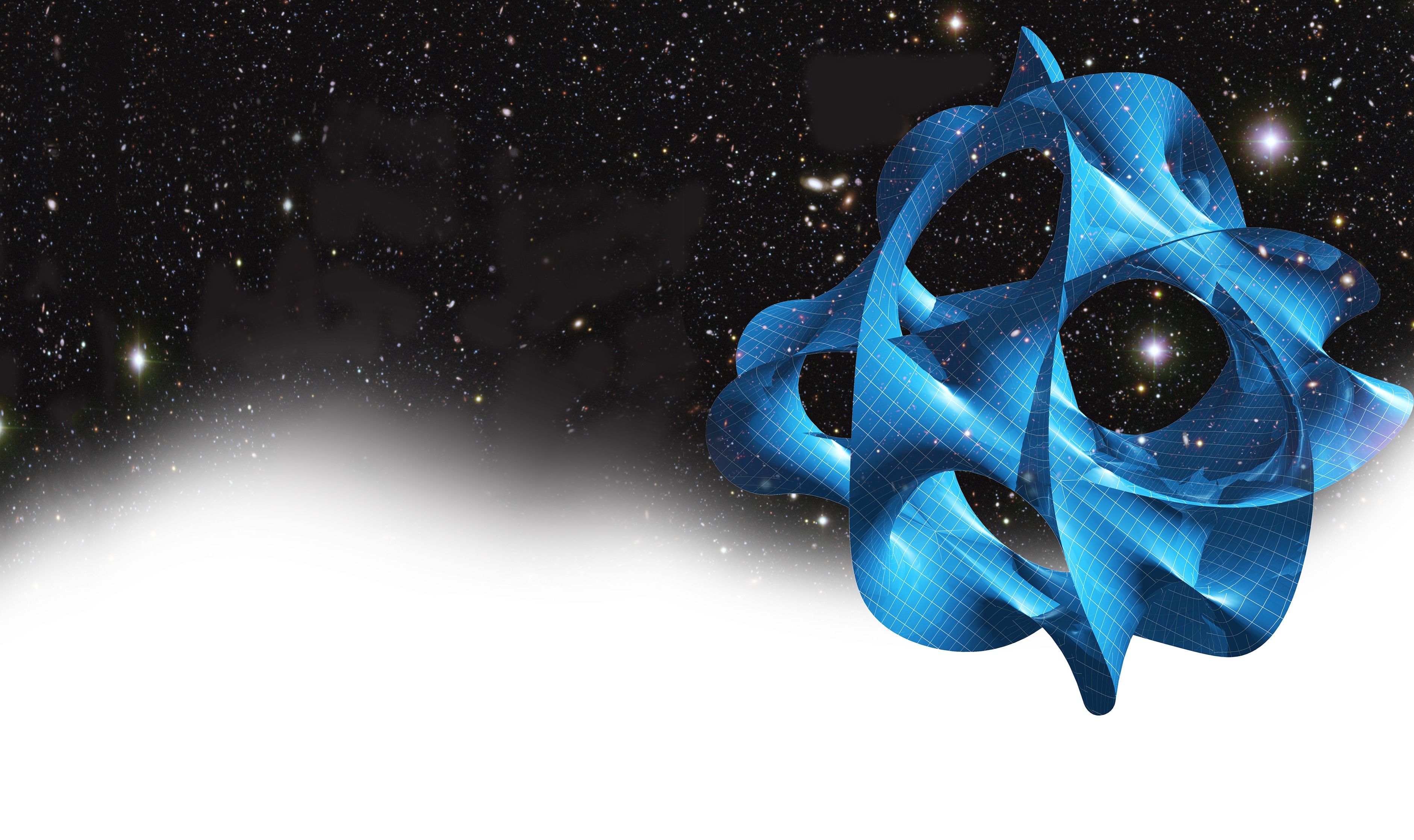
String theory’s elegance masks a formidable mathematical complexity. Its framework demands a deep understanding of concepts far beyond the typical physicist’s toolkit, requiring mastery of advanced techniques from differential geometry, topology, and algebraic geometry, often pushing the boundaries of known mathematics. The sheer intricacy of the calculations involved often necessitates the use of powerful computational tools, highlighting the symbiotic relationship between theoretical physics and advanced computational methods in this field.The mathematical landscape of string theory is vast and multifaceted.
Different formulations, or versions, exist, each offering a unique perspective and posing distinct mathematical challenges. These variations arise from different choices in compactification (how extra dimensions are curled up) and the specific types of string interactions considered. The most prominent versions include Type I, Type IIA, Type IIB, and the five different superstring theories, all potentially connected through a conjectured overarching theory known as M-theory.
These different versions are not simply different ways of describing the same underlying physics, but rather represent distinct theoretical frameworks, each with its own mathematical structure and implications.
Comparison of String Theory Formulations
The various formulations of string theory, while seemingly disparate, are believed to be connected through a web of dualities. These dualities reveal unexpected relationships between different theories, suggesting that they might be different facets of a single, more fundamental theory. For example, T-duality relates string theories compactified on circles of different radii, while S-duality connects weak and strong coupling regimes.
Understanding these dualities is crucial for unraveling the complete mathematical structure of string theory and its implications for our understanding of the universe. The mathematical tools used to explore these dualities often involve advanced concepts from conformal field theory and mirror symmetry.
Recent Advancements in String Theory Mathematics
Significant progress has been made in recent years in understanding the mathematical underpinnings of string theory. Advances in areas such as mirror symmetry have provided powerful new techniques for calculating string theory amplitudes. Mirror symmetry, a surprising duality between Calabi-Yau manifolds, allows for the computation of seemingly intractable integrals by mapping them to simpler, related problems. This has led to breakthroughs in understanding the low-energy effective theories that emerge from string theory, providing valuable insights into potential connections with observed physics.
Furthermore, developments in topological string theory have offered new perspectives on the non-perturbative aspects of string theory, a domain where traditional perturbative methods are insufficient. These advancements are not merely theoretical curiosities; they offer potential pathways to make testable predictions and to ultimately connect string theory to experimental observations.
Challenges in String Theory’s Mathematical Framework
Despite significant progress, formidable mathematical challenges remain. The non-perturbative aspects of string theory, where the effects of strong interactions are crucial, are still poorly understood. Many calculations rely on perturbative approximations, which are only valid in certain regimes. Developing reliable non-perturbative methods is essential for a complete understanding of string theory. Furthermore, the vast landscape of possible string vacua—the multitude of different solutions to the equations of string theory—presents a significant hurdle.
Identifying the specific vacuum that corresponds to our universe remains a major challenge. The mathematical complexity associated with navigating this landscape and selecting a physically realistic vacuum represents a significant obstacle to further progress. The lack of a complete and self-consistent mathematical formulation remains a key challenge for string theory.
Alternative Theories and Competing Paradigms
The dominance of string theory in the quest for a unified theory of physics has spurred the development of alternative approaches to quantum gravity. These competing frameworks offer distinct perspectives on the fundamental nature of spacetime, gravity, and the universe, each with its own strengths and weaknesses. Examining these alternatives provides a crucial context for evaluating string theory’s progress and limitations.
Detailed Overview of Competing Theoretical Frameworks
The following table summarizes five prominent alternative theoretical frameworks to string theory, focusing on their core postulates, key concepts, and mathematical formalisms. Each framework attempts to reconcile general relativity and quantum mechanics, but employs fundamentally different methods.
Theory Name | Core Postulates | Key Concepts | Mathematical Formalism |
---|---|---|---|
Loop Quantum Gravity (LQG) | Spacetime is fundamentally granular, composed of loops of quantized geometry. Gravity emerges from the quantum geometry of spacetime. | Spin networks, spin foams, area and volume operators. | Uses techniques from non-commutative geometry and topological quantum field theory. Key mathematical structures include spin networks and spin foams, represented by graphs and their evolution. No single unifying equation exists, but the dynamics are described through path integrals over spin foams. |
Causal Set Theory | Spacetime is a discrete structure, a partially ordered set of events (causal set) reflecting the causal relations between events. | Causal relations, discrete spacetime, order structure. | Uses combinatorial and order-theoretic methods. The fundamental object is a partially ordered set, and the dynamics are defined by probabilistic processes on these sets. |
Asymptotic Safety | Quantum gravity is a non-perturbatively renormalizable theory, meaning its infinities can be controlled without the need for extra dimensions or supersymmetry. | Renormalization group flow, fixed points, non-perturbative methods. | Relies heavily on the renormalization group equations, particularly the study of fixed points in the theory’s space of couplings. |
Superfluid Vacuum Theory | Spacetime emerges from a superfluid quantum vacuum, with gravity arising from its collective excitations. | Superfluid vacuum, collective excitations, emergent spacetime. | Uses techniques from condensed matter physics and quantum field theory. The mathematical formalism involves effective field theories describing the superfluid vacuum and its excitations. |
Emergent Gravity | Gravity is not a fundamental force but rather an emergent phenomenon arising from the collective behavior of microscopic degrees of freedom. Different approaches exist, including those rooted in entanglement entropy. | Entanglement, microscopic degrees of freedom, emergent spacetime. | The mathematical formalism varies significantly depending on the specific realization of emergent gravity, ranging from tensor networks to holographic approaches. |
Strengths and Weaknesses Compared to String Theory
The following table compares the five alternative theories to string theory, focusing on their ability to address key issues in theoretical physics.
Theory Name | Strengths (relative to string theory) | Weaknesses (relative to string theory) | Areas of Superiority |
---|---|---|---|
Loop Quantum Gravity | Background-independent, potential for testable predictions related to black hole thermodynamics and the early universe. | Lack of a complete quantum theory of gravity, difficulty in incorporating the Standard Model. | More direct approach to quantization of spacetime. |
Causal Set Theory | Elegant framework for discrete spacetime, potentially solves the singularity problem. | Limited predictive power, challenges in connecting to observations. | Simplicity and conceptual clarity. |
Asymptotic Safety | Potential for a UV-complete theory of quantum gravity without requiring extra dimensions or supersymmetry. | Still under development, limited predictive power at present. | Potential for a more minimalistic approach to quantum gravity. |
Superfluid Vacuum Theory | Connects gravity to condensed matter physics, potentially offers new avenues for experimental tests. | Theoretical challenges in explaining the detailed properties of spacetime. | Potential for novel experimental predictions. |
Emergent Gravity | Addresses the problem of unifying gravity with other forces from a different perspective. | Diverse approaches with varying degrees of development and testability. | Potential for a more fundamental understanding of gravity’s origin. |
Power and Predictive Capabilities
Each alternative theory offers a unique approach to explaining existing data and making testable predictions. However, their current experimental status varies significantly.Loop Quantum Gravity predicts deviations from general relativity in strong gravitational fields, potentially observable in gravitational wave observations or through precision measurements of black hole properties. Experimental verification remains a significant challenge.Causal Set Theory currently lacks direct observational consequences, with future development focusing on finding connections to cosmological observations or developing testable predictions.Asymptotic Safety predicts specific features of the renormalization group flow that could be tested through lattice simulations or through high-energy experiments, though these are highly challenging.Superfluid Vacuum Theory offers potentially testable predictions related to the properties of the vacuum, potentially observable through precision measurements of cosmological constants or gravitational wave polarization.Emergent Gravity, due to its diverse approaches, has varied predictive capabilities depending on the specific model.
Some versions offer testable predictions related to black hole thermodynamics or the early universe, while others are more focused on conceptual advancements.
While string theory offers a mathematically elegant framework, its lack of testable predictions currently hinders its empirical validation. Conversely, Loop Quantum Gravity shows promise with its predictions regarding black hole thermodynamics, though further research is needed to solidify its power and resolve outstanding theoretical challenges. Similarly, the potential experimental signatures of Superfluid Vacuum Theory warrant further investigation.
Further Considerations
Loop Quantum Gravity challenges our understanding of spacetime by proposing a discrete structure, impacting our notions of causality and the very nature of space and time. Open problems include finding a consistent way to incorporate the Standard Model and developing more concrete experimental predictions.Causal Set Theory presents a fundamentally different view of spacetime as a discrete causal structure, potentially altering our perception of continuity and the arrow of time.
A major open problem is connecting the theory to observations and developing testable predictions.Asymptotic Safety offers a path towards a UV-complete quantum gravity without the need for extra dimensions or supersymmetry, potentially simplifying our understanding of fundamental physics. However, developing robust non-perturbative methods and deriving specific testable predictions remain major challenges.Superfluid Vacuum Theory connects gravity to condensed matter physics, suggesting a new perspective on the nature of spacetime and its relationship to matter.
However, more theoretical work is needed to explain the details of spacetime emergence and make precise experimental predictions.Emergent Gravity offers a radical departure from traditional views of gravity, suggesting that gravity is not a fundamental force but an emergent phenomenon. The open problems are vast and depend on the specific approach; many lack well-defined predictions and require further development.
String Theory’s Predictions and Observational Consequences
String theory, despite its elegance and mathematical sophistication, faces a significant challenge: a lack of direct experimental verification. While many of its predictions lie far beyond the reach of current technology, some offer potential avenues for future investigation, primarily in particle physics and cosmology. These predictions, while not definitive confirmations, provide testable hypotheses that could significantly advance our understanding of the universe’s fundamental structure.
The following analysis explores several key predictions, their testability, and the current status of experimental efforts.
Predictions from String Theory and Their Experimental Testability
The following table summarizes specific predictions from string theory, their testability, current experimental status, and the underlying theoretical framework. It’s crucial to remember that many predictions depend on specific model-building choices, making the connection between theory and observation complex.
Prediction | Testability | Current Status | Theoretical Framework |
---|---|---|---|
Existence of Supersymmetric Particles | Detection of supersymmetric partners at the Large Hadron Collider (LHC) or future colliders with higher energy. Requires precise measurements of particle masses and interactions. | The LHC has not yet found evidence for supersymmetry, placing constraints on certain supersymmetric models. Future colliders with higher energy and luminosity are needed. The non-observation of supersymmetry at the LHC has weakened, but not eliminated, support for many string theory models that require it. | Most string theory models incorporate supersymmetry to solve theoretical problems like the hierarchy problem. Supersymmetry breaking mechanisms are crucial for making contact with the observed world. |
Specific patterns of Yukawa couplings | Precise measurements of particle masses and their interactions at high-energy colliders. This requires extremely high precision measurements and sophisticated data analysis techniques. | Current data from the LHC provides some constraints but not sufficient precision to confirm or refute specific predictions. Future experiments with higher precision are needed. While some patterns hinted at, they remain inconclusive. | Specific compactification schemes in string theory can lead to predictable patterns in the Yukawa couplings, which determine the strength of interactions between particles. |
Gravitational Waves with Specific Polarization | Detection of gravitational waves with non-tensorial polarizations using advanced gravitational wave detectors like LIGO/Virgo and future space-based detectors like LISA. | Current gravitational wave observations are consistent with general relativity, but haven’t ruled out the possibility of additional polarizations predicted by some string theory models. Future detectors with improved sensitivity are essential. | Some string theory models predict the existence of additional gravitational wave polarizations beyond those predicted by general relativity. |
Moduli Fields and Their Effects on Cosmology | Precise measurements of the cosmic microwave background (CMB) and large-scale structure of the universe. Requires highly sensitive observations and sophisticated cosmological models. | Current CMB data provides some constraints on certain moduli fields, but more precise data and theoretical understanding are needed. Future CMB experiments like CMB-S4 could offer more stringent tests. | String theory predicts the existence of moduli fields, which can affect the early universe’s evolution and leave observable imprints on the CMB and large-scale structure. |
Extra spatial dimensions and their signatures at high energies | Observation of deviations from the inverse-square law of gravity at short distances, or the production of Kaluza-Klein particles at high-energy colliders. | Current experimental limits constrain the size and nature of extra dimensions. Experiments searching for deviations from inverse-square law gravity are ongoing, with null results so far. Future experiments at higher energy scales could reveal evidence. | Many string theory models predict the existence of extra spatial dimensions compactified at small scales. These dimensions could manifest themselves through deviations from known physics at high energies. |
Implications of Confirming or Refuting Predictions
The confirmation of any of the predictions listed above would represent a major breakthrough for string theory. The detection of supersymmetric particles, for instance, would provide strong evidence for the underlying framework of many string theory models. Conversely, the continued absence of such particles would severely constrain or even rule out many of these models, necessitating a re-evaluation of the theoretical framework.
Similarly, the detection of specific gravitational wave polarizations would strongly support certain string theory models, while the absence of such polarizations would again challenge the theory. The observation of extra spatial dimensions would revolutionize our understanding of spacetime. In contrast, a failure to observe any of these phenomena would not necessarily invalidate string theory entirely, but it would necessitate significant modifications or alternative interpretations.
Challenges in Testing String Theory Predictions: The primary challenge lies in the extremely high energy scales involved. String theory effects are predicted to become apparent at the Planck scale (around 1019 GeV), far beyond the reach of current and foreseeable particle accelerators. Furthermore, theoretical ambiguities remain, particularly regarding the specific compactification schemes and supersymmetry breaking mechanisms. These ambiguities lead to a vast landscape of possible string theory models, making it difficult to make precise, falsifiable predictions. Experimental limitations, such as the precision of measurements and the background noise in experiments, also pose significant obstacles.
Finally, the interpretation of experimental results within the context of string theory is complicated by the need to bridge the gap between the high-energy scales of the theory and the low-energy scales of observable phenomena.
The Role of String Theory in Cosmology
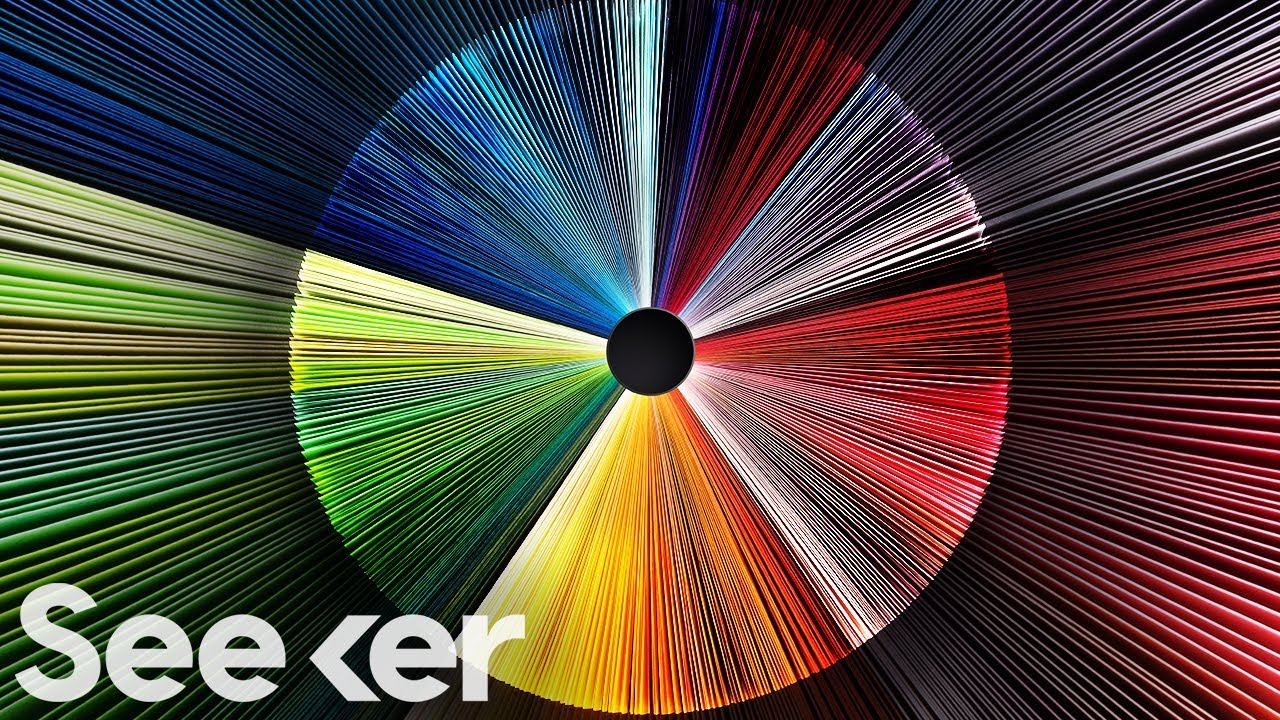
String theory, with its elegant framework of fundamental vibrating strings and extra spatial dimensions, offers a potentially revolutionary perspective on cosmology, addressing some of the most profound mysteries of the universe’s origin and evolution. While still largely theoretical, its implications for cosmological phenomena are far-reaching, prompting significant investigation and debate within the scientific community. This exploration delves into the specific applications and implications of string theory within the cosmological context.
Singularity Resolution at the Big Bang
String theory proposes to resolve the Big Bang singularity, the point of infinite density and temperature at the universe’s beginning, through several mechanisms. Crucially, the introduction of extra spatial dimensions plays a pivotal role. In brane cosmology, our universe is considered a three-dimensional brane embedded within a higher-dimensional spacetime. The Big Bang singularity might then be an artifact of our limited four-dimensional perspective; the higher-dimensional spacetime could have remained smooth and well-behaved even as our brane experienced a “Big Bang.” This contrasts with alternative singularity resolution models, such as loop quantum gravity, which attempt to resolve the singularity through a quantization of spacetime itself, resulting in a “bounce” rather than a singularity.
The key difference lies in the fundamental nature of the resolution: string theory modifies the geometry through extra dimensions, while loop quantum gravity modifies the very fabric of spacetime.
Cosmological Constant and the Landscape of Vacua
String theory’s vast “landscape” of possible vacua—different stable configurations of the theory—offers a potential explanation for the observed cosmological constant, the energy density associated with dark energy. Each vacuum possesses a different value for the cosmological constant, and the observed value might simply reflect the particular vacuum our universe happens to inhabit. The anthropic principle, suggesting that only universes with properties conducive to life will be observed, plays a significant role in this interpretation.
However, this approach faces limitations; predicting the probability distribution of vacua and thus the likelihood of our observed cosmological constant remains a significant challenge.
Vacuum Type | Description | Predicted Cosmological Constant (Units of Planck Density) |
---|---|---|
Type I | Open string theory with gauge group SO(32) | ~10-120 (Illustrative, highly model-dependent) |
Heterotic SO(32) | Heterotic string theory with gauge group SO(32) | ~10-123 (Illustrative, highly model-dependent) |
Heterotic E8xE8 | Heterotic string theory with gauge group E8xE8 | ~10-122 (Illustrative, highly model-dependent) |
Primordial Density Fluctuations
String theory offers mechanisms for generating the primordial density fluctuations that seeded the large-scale structure of the universe. These fluctuations are believed to have originated in the very early universe and are responsible for the distribution of galaxies and galaxy clusters we observe today. While inflationary cosmology provides a successful framework for generating these fluctuations, string theory offers alternative mechanisms, potentially involving processes occurring in the extra dimensions or interactions with branes.
The power spectrum of density fluctuations—a measure of the amplitude of fluctuations at different scales—provides a crucial observational test for both inflationary and string-theoretic models. A hypothetical power spectrum diagram would show the amplitude of fluctuations as a function of wavenumber (or scale), exhibiting characteristic features that could distinguish between different models.
The question “Is string theory dead?” sparks debate. To understand the difference between declaring it defunct and simply acknowledging its limitations, we must first grasp the crucial distinction between a scientific theory and a hypothesis; consider the key differences outlined in this insightful resource: which of the following distinguishes a theory from a hypothesis. Ultimately, whether string theory thrives or fades depends on future experimental verification, not mere speculation.
Addressing the Horizon Problem
String theory offers potential mechanisms to address the horizon problem, the observation that seemingly causally disconnected regions of the universe exhibit remarkable homogeneity. In standard cosmology, this homogeneity is difficult to explain, as regions separated by distances greater than the particle horizon (the maximum distance light could have traveled since the Big Bang) could not have interacted. String theory, through its extra dimensions or other mechanisms, might have allowed for a period of faster-than-light communication or interaction in the early universe, thus establishing the observed homogeneity.
“The observed homogeneity of the CMB [Cosmic Microwave Background] requires a mechanism to establish causal contact between widely separated regions in the early universe. String theory offers a potential solution through the interaction of branes or other mechanisms that may have existed in the early universe.” — Hypothetical excerpt from a seminal paper.
Unified Description of Inflation and Dark Energy
String theory holds the potential to provide a unified description of inflation and dark energy, connecting the early universe’s inflationary epoch with the late-time acceleration. Models that link the dynamics of extra dimensions or the evolution of the string theory landscape to both inflation and dark energy are being actively explored. However, observationally testing these models presents significant challenges, requiring extremely precise cosmological data and a deep understanding of the underlying string theory mechanisms.
Addressing the Flatness Problem
The flatness problem refers to the observation that the universe’s geometry is remarkably close to flat, despite the fact that small deviations from flatness would have been amplified over cosmic time. String theory, through mechanisms such as brane-world scenarios or modifications to gravity at very high energies, offers potential solutions to this problem. These mechanisms differ from other solutions within the standard cosmological model, such as inflation, which also addresses the flatness problem by stretching the universe to a near-flat state.
Evolution from the Planck Epoch to Nucleosynthesis
String theory makes predictions for the universe’s evolution from the Planck epoch (the earliest moments after the Big Bang) to the era of nucleosynthesis (when light elements formed). These predictions involve specific scenarios for the evolution of extra dimensions, the breaking of supersymmetry, and the production of particles. Observational consequences, such as subtle variations in the abundances of light elements or characteristic signatures in the cosmic microwave background, could potentially test these predictions.
Supersymmetry Breaking and Dark Matter
Supersymmetry breaking, a crucial aspect of many string theory models, plays a significant role in string cosmology. Different supersymmetry breaking mechanisms lead to distinct cosmological consequences, influencing the evolution of the early universe and the generation of dark matter.
Supersymmetry Breaking Mechanism | Cosmological Implications |
---|---|
Moduli Stabilization | Specific particle spectrum, potential impact on inflation |
Gaugino Condensation | Generation of dark matter candidates |
Anomaly Mediation | Effects on the early universe expansion rate |
Observational Tests of String Theory in Cosmology
Future astronomical observations, particularly those related to gravitational waves and cosmic microwave background anisotropies, offer potential avenues for testing string theory’s cosmological predictions. Specific signatures in the polarization of the CMB or the detection of primordial gravitational waves with specific properties could provide evidence for or against certain string-theoretic scenarios. The detection of specific particle signatures from the early universe, predicted by some string theory models, could also serve as a crucial test.
String Theory and Quantum Gravity
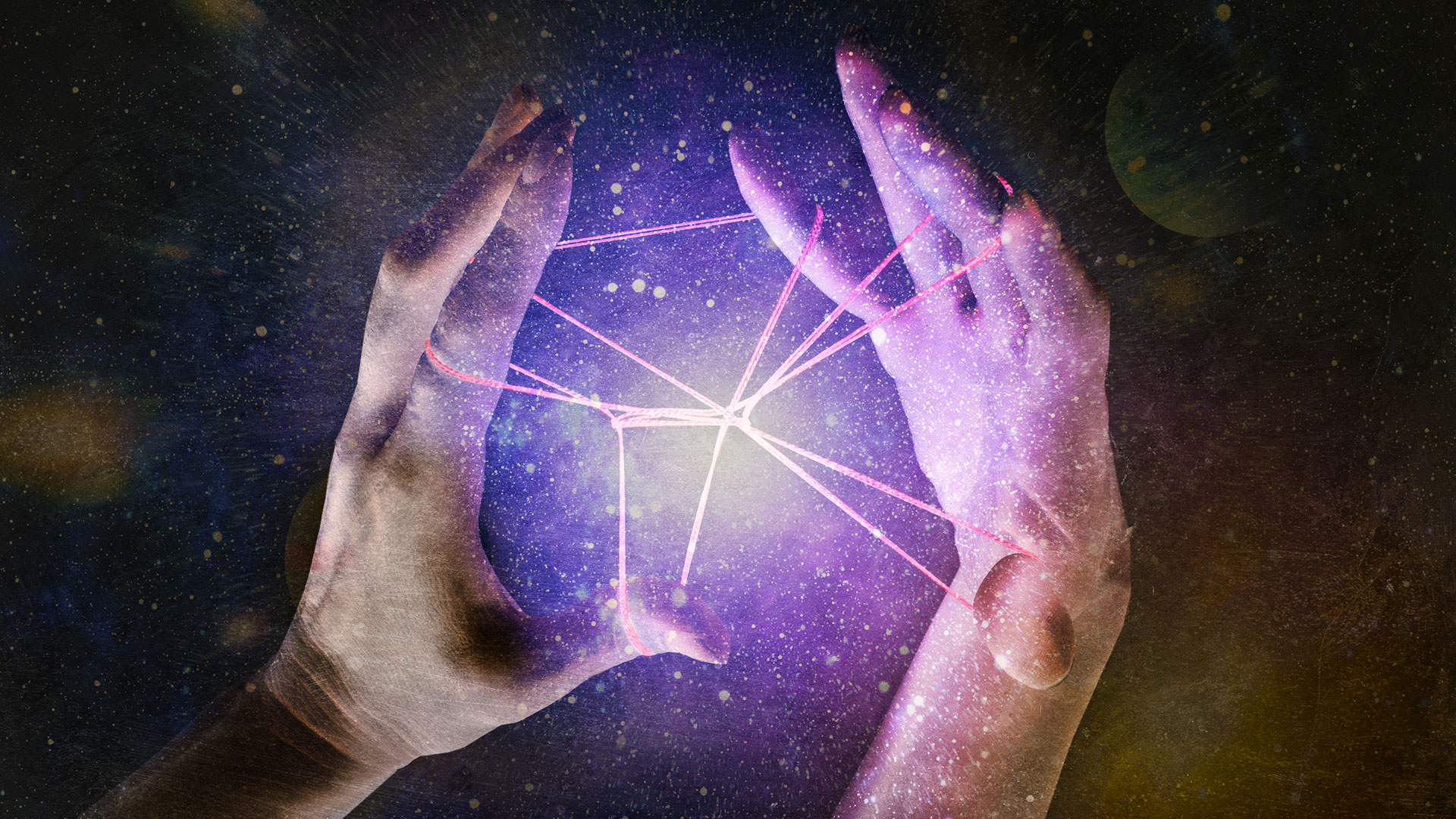
String theory occupies a unique position in theoretical physics, offering a potential framework for unifying two seemingly incompatible pillars of modern physics: quantum mechanics and general relativity. The incompatibility arises from the fact that general relativity, describing gravity at macroscopic scales, is a classical theory, while quantum mechanics governs the realm of the very small. String theory attempts to resolve this conflict by proposing a fundamental shift in our understanding of the universe’s building blocks.String theory postulates that fundamental particles are not point-like objects, but rather tiny, vibrating strings.
The different vibrational modes of these strings correspond to different particles, including gravitons, the hypothetical force-carrying particles of gravity. This inherently incorporates gravity into the quantum framework, offering a path towards a theory of quantum gravity. The theory’s elegance lies in its ability to potentially reconcile the seemingly irreconcilable, offering a unified description of all fundamental forces and matter.
Comparison of String Theory and Other Quantum Gravity Approaches
String theory is not the only contender in the quest for quantum gravity. Loop quantum gravity, for example, takes a different approach, focusing on quantizing the fabric of spacetime itself rather than the fundamental particles. Causal set theory offers yet another perspective, suggesting that spacetime is fundamentally discrete. Each of these theories faces unique mathematical and conceptual challenges.
While string theory utilizes a framework rooted in higher-dimensional geometry and supersymmetry, loop quantum gravity employs techniques from mathematical topology and non-commutative geometry. Causal set theory, on the other hand, emphasizes the causal structure of spacetime.
Key Differences and Similarities Between Approaches
A significant difference lies in their fundamental objects. String theory uses vibrating strings, loop quantum gravity uses loops of quantized spacetime, and causal set theory uses discrete causal sets as fundamental building blocks. Despite these differences, there are also some shared goals. All three aim to provide a consistent and complete theory of quantum gravity, capable of describing the universe at all scales, from the subatomic to the cosmological.
Furthermore, all three face the significant challenge of making testable predictions that could distinguish them from each other and from existing theories. The search for experimental verification remains a central hurdle for all approaches to quantum gravity. Each theory offers a unique mathematical language and conceptual framework for tackling this profound problem.
The Landscape of String Theory Solutions: Is String Theory Dead
String theory, in its various formulations, possesses a staggering number of possible solutions, far exceeding the single, unique universe we observe. This vastness, often referred to as the “string theory landscape,” presents both a remarkable opportunity and a significant challenge to the theory’s predictive power. Understanding this landscape and navigating its complexities is crucial for determining whether string theory can truly provide a fundamental description of our reality.The sheer number of solutions arises from the intricate ways in which various parameters, such as the compactification of extra spatial dimensions and the values of coupling constants, can be configured.
These parameters determine the low-energy effective theories that emerge from the underlying string dynamics, resulting in a multitude of possible universes, each with potentially distinct physical laws and properties. This diversity, while initially daunting, reflects the theory’s flexibility in accommodating a wide range of possibilities, including potentially our own universe. The challenge, however, lies in identifying the specific solution—or solutions—that accurately describes our observable universe.
The Implications of the Landscape for Predictability
The existence of the string theory landscape raises serious concerns regarding the theory’s predictive power. If there are countless possible universes, each with different physical constants and laws, how can string theory make specific, testable predictions about our universe? This is a significant hurdle, as the ability to make precise predictions is a hallmark of a successful scientific theory.
The landscape itself doesn’t directly dictate which solution corresponds to our universe; it only highlights the vast array of possibilities. This lack of a unique solution poses a challenge to the falsifiability of the theory, a key tenet of the scientific method. For example, if a particular prediction fails to match observation, one could simply argue that our universe corresponds to a different point in the landscape.
Methods for Constraining the Landscape
Despite the challenges, considerable effort is being devoted to constraining the landscape and identifying preferred solutions. Researchers are exploring various avenues to narrow down the possibilities. One approach involves imposing physical constraints based on our observations of the universe, such as the values of fundamental constants, the existence of specific particles, or the cosmological evolution of the universe. Another strategy involves developing more refined mathematical techniques to classify and analyze the vast number of solutions, searching for patterns and organizing principles that might reveal preferred regions within the landscape.
Furthermore, ongoing developments in computational methods are enabling more extensive explorations of the landscape, allowing researchers to systematically search for solutions that match observational data. For example, specific values for the cosmological constant, a key parameter in cosmology, might be used to filter the landscape and select potentially viable solutions. These approaches aim to move beyond simply cataloging solutions and toward identifying those that are physically plausible and consistent with our observations.
Impact on Other Areas of Physics
String theory, despite its focus on quantum gravity and cosmology, has surprisingly exerted a significant influence on various other branches of physics. Its mathematical tools and conceptual frameworks have proven surprisingly adaptable, enriching our understanding and offering new avenues for research in seemingly unrelated fields. This section will explore these impacts, highlighting both successes and limitations.
Specific Areas of Influence
String theory’s influence extends beyond its primary goals. The intricate mathematical structures and novel concepts it employs have found unexpected applications in diverse areas of physics. These applications often involve the adaptation of string-theoretic techniques to problems in other fields, rather than a direct application of string theory itself as a fundamental theory of nature.
- Condensed Matter Physics (Topological Insulators): String-theoretic techniques, particularly those related to topological field theories, have aided in the classification and understanding of topological insulators, exotic materials with unique electronic properties.
- Nuclear Physics (Quark-Gluon Plasma): String-inspired models have been used to describe the behavior of quark-gluon plasma, a state of matter believed to have existed in the early universe, offering insights into its properties and dynamics.
- Fluid Dynamics (Turbulence): Certain aspects of string theory, particularly the AdS/CFT correspondence, have been applied to understand the complex behavior of turbulent fluids, offering new mathematical tools for analysis.
- Quantum Information Theory (Quantum Computing): Concepts from string theory, particularly those related to entanglement and quantum field theory, have found applications in quantum information theory and the development of quantum computing algorithms.
- Statistical Mechanics (Critical Phenomena): The mathematical framework of string theory, particularly conformal field theory, has provided valuable tools for understanding critical phenomena in statistical mechanics, such as phase transitions.
Detailed Examples of String Theory’s Impact
The following table provides specific examples of string theory’s influence across various areas of physics.
Area of Physics | Specific String Theory Concept Applied | Example of Application | Reference |
---|---|---|---|
Condensed Matter Physics (Topological Insulators) | Topological Field Theory | Classification of topological insulators based on topological invariants derived from string theory techniques. | [Citation needed: A relevant review article on topological insulators and string theory would be appropriate here.] |
Nuclear Physics (Quark-Gluon Plasma) | Gauge/Gravity Duality (AdS/CFT) | Modeling the strongly coupled dynamics of quark-gluon plasma using holographic techniques derived from the AdS/CFT correspondence. | [Citation needed: A reputable article on AdS/CFT applications to quark-gluon plasma] |
Fluid Dynamics (Turbulence) | Conformal Field Theory | Applying conformal field theory techniques to analyze the scaling properties of turbulent flows. | [Citation needed: A relevant article on CFT and turbulence] |
Quantum Information Theory (Quantum Computing) | Quantum Field Theory techniques | Developing new quantum algorithms inspired by the mathematical structures of quantum field theories related to string theory. | [Citation needed: A reputable source on quantum computing and string theory-inspired algorithms] |
Statistical Mechanics (Critical Phenomena) | Conformal Field Theory | Using conformal field theory to calculate critical exponents and other properties of phase transitions. | [Citation needed: A relevant review article on CFT and critical phenomena] |
Potential Future Applications – A Predictive Analysis
Within the next 20 years, we can anticipate significant advancements in the application of string-theoretic concepts to condensed matter physics and quantum information theory. The development of more powerful quantum computers, coupled with a deeper understanding of topological materials, will likely facilitate the design of novel quantum devices inspired by string-theoretic models. For example, the development of fault-tolerant quantum computers could allow for the simulation of complex string-theoretic models, leading to new insights into the behavior of condensed matter systems and the development of more efficient quantum algorithms. Similarly, the ongoing research into topological quantum computation, which draws heavily from topological field theories closely related to string theory, could lead to the creation of quantum computers that are inherently robust against noise and decoherence. These advancements would be greatly facilitated by improvements in material science and nanotechnology.
Limitations and Challenges
The application of string theory to other areas of physics faces several significant limitations and challenges:
- Mathematical Complexity: The mathematical formalism of string theory is notoriously complex, making it difficult to apply to specific physical problems.
- Lack of Experimental Verification: The absence of direct experimental verification of string theory makes it challenging to assess its validity and applicability in other fields.
- Conceptual Gaps: Bridging the gap between the abstract mathematical structures of string theory and the concrete physical phenomena in other areas requires significant theoretical developments.
- Computational Limitations: Solving the complex equations arising from string theory applications often requires significant computational resources, which can be a bottleneck.
Comparative Analysis
Compared to alternative frameworks like loop quantum gravity, string theory’s impact on other areas of physics is arguably broader, primarily due to the rich mathematical toolbox it offers. While loop quantum gravity focuses more directly on the quantization of spacetime, string theory’s influence is felt in various fields through its mathematical tools, such as topological field theories and conformal field theories.
For example, in condensed matter physics, string-inspired topological field theories provide a powerful framework for classifying topological phases of matter, an area where loop quantum gravity has had less impact. However, loop quantum gravity’s focus on background-independent quantization may offer advantages in certain cosmological contexts, where string theory’s reliance on background metrics could be a limitation. The choice between these frameworks depends on the specific problem being addressed.
String Theory’s Impact: A Summary Essay
String theory, despite its initial focus on resolving the incompatibility between general relativity and quantum mechanics, has profoundly impacted diverse areas of physics beyond its core goals. Its influence stems not only from its potential to unify fundamental forces but also from its rich mathematical framework and the novel concepts it introduces. This essay will explore the successes and limitations of string theory’s impact on condensed matter physics, nuclear physics, fluid dynamics, quantum information theory, and statistical mechanics.One of the most significant contributions of string theory lies in its provision of powerful mathematical tools.
Topological field theories, a cornerstone of string theory, have been instrumental in classifying topological insulators in condensed matter physics, materials exhibiting unique electronic properties with potential for revolutionary technological applications. Similarly, techniques derived from the AdS/CFT correspondence, a key concept within string theory, have been applied to model the complex dynamics of quark-gluon plasma in nuclear physics, providing valuable insights into this exotic state of matter.
In fluid dynamics, conformal field theory, another essential component of string theory, has offered new approaches to understanding turbulence, a notoriously challenging problem. Furthermore, concepts related to quantum field theory, deeply intertwined with string theory, have found applications in quantum information theory, potentially influencing the development of more efficient quantum algorithms. Finally, the mathematical structure of string theory has also provided valuable tools for understanding critical phenomena in statistical mechanics, improving our ability to model phase transitions.However, the application of string theory is not without limitations.
The mathematical complexity of string theory poses a significant challenge, often requiring substantial computational resources and making it difficult to apply to specific physical problems. The lack of direct experimental verification further hinders its widespread acceptance and application. Moreover, bridging the gap between the abstract mathematical structures of string theory and the concrete physical phenomena in other fields requires significant theoretical developments.
The computational demands of applying string theory to complex systems also pose a considerable barrier.In comparison to other theoretical frameworks, such as loop quantum gravity, string theory’s impact on these diverse fields appears broader. While loop quantum gravity focuses on quantizing spacetime, string theory’s mathematical tools have found wider applications. However, both frameworks face significant challenges, and the ultimate success of either in unifying fundamental physics remains an open question.
Despite these limitations, the contributions of string theory to various areas of physics are undeniable, highlighting its potential as a source of new mathematical techniques and conceptual insights. Future advancements in computational power and experimental techniques may further unlock the potential of string theory, leading to even more profound impacts on our understanding of the physical world.
The “Dead” Metaphor and its Interpretations
The declaration of string theory’s “death” is a recurring theme in popular science discussions, often fueled by the lack of experimental verification and the theory’s inherent complexities. However, the metaphor itself is a gross oversimplification of a nuanced and ongoing scientific debate. The vitality of string theory isn’t a binary on/off switch; it’s a spectrum of perspectives reflecting the theory’s current state and the diverse approaches of physicists working within its framework.The term “dead,” when applied to string theory, typically reflects frustration with the lack of empirical evidence directly supporting its predictions.
Many physicists, particularly those working in experimental fields, view the absence of verifiable experimental results as a significant impediment, leading them to question the theory’s usefulness and to explore alternative avenues. This perspective often translates to a perception of string theory as a stagnant field, unproductive in terms of testable predictions and tangible advancements in our understanding of the universe.
Different Interpretations of the “Dead” Metaphor
The “death” of string theory is not a universally accepted conclusion. A significant portion of the theoretical physics community remains actively engaged with string theory, pursuing various avenues of research. These researchers often view the “dead” metaphor as premature and overly dramatic. They emphasize the theory’s mathematical elegance and its potential to unify quantum mechanics and general relativity, two pillars of modern physics that remain irreconcilably disparate within the standard model.
They see ongoing mathematical developments and explorations of its cosmological implications as signs of life and continuing potential. The disagreement stems from differing priorities: the experimentalists’ focus on empirical validation versus the theorists’ emphasis on mathematical consistency and conceptual unification.
The Nuances of the Ongoing Debate
The debate surrounding string theory’s vitality is not merely a disagreement about its current state, but also reflects deeper philosophical differences within the physics community. Some physicists argue that a theory’s value lies primarily in its ability to make testable predictions and explain observed phenomena. Others prioritize mathematical consistency and elegance, believing that a beautiful and internally consistent theory is more likely to be correct, even if its predictions are currently beyond our experimental reach.
This tension highlights the inherent challenges in evaluating theoretical frameworks, particularly those dealing with phenomena far beyond our current experimental capabilities, like quantum gravity at the Planck scale. The ongoing research in string theory, therefore, is not simply a search for experimental confirmation, but also a process of refining the theory itself and exploring its implications across various branches of physics.
The “dead” metaphor fails to capture this multifaceted reality.
Funding and Research in String Theory
The financial landscape of string theory research has been, and continues to be, a complex interplay of scientific merit, political priorities, and economic realities. Funding levels have fluctuated significantly over the decades, reflecting changing perceptions of the field’s potential and the broader scientific priorities of funding agencies. Understanding these funding trends is crucial to grasping the evolution of string theory itself.Funding levels for string theory research are not easily quantified with a single, universally accepted metric.
Data is often dispersed across various funding agencies and research institutions, and categorizing projects solely as “string theory” can be challenging due to the field’s interdisciplinary nature. However, we can glean insights from available data and expert opinions to sketch a general picture.
The question, “Is string theory dead?” remains a lively debate among physicists. For comprehensive, cutting-edge information on complex scientific theories, explore the vast resources available at the genesys knowledge base ; it’s a treasure trove for researchers. Ultimately, whether string theory’s future is bright or dim depends on continued exploration and innovative thinking.
Funding Trends in String Theory Research
A precise timeline requires detailed, aggregated data from numerous sources, which is not readily available in a consolidated form. However, we can describe general trends. The initial surge of interest and funding in the 1980s, following the first string revolution, was substantial, leading to a significant increase in research positions and dedicated projects. This period saw considerable investment from both government agencies (like the National Science Foundation in the US and equivalent agencies in other countries) and private foundations.
The subsequent decades saw a period of relatively stable, though perhaps less exuberant, funding. However, the rate of growth in funding likely did not keep pace with the overall growth of scientific research budgets, leading to a perceived (though not necessarily absolute) decrease in the relative proportion of funding dedicated to string theory compared to other areas of physics.
More recent years have seen a degree of fluctuation, with funding influenced by factors discussed below.
Factors Influencing Funding Levels
Several factors significantly influence the level of funding allocated to string theory research. These include:
- Scientific breakthroughs and potential applications: Major theoretical advances or the promise of tangible applications (e.g., in condensed matter physics or quantum computing) can lead to increased funding. Conversely, periods without significant breakthroughs may result in reduced funding.
- Competition with other scientific fields: Funding agencies have limited resources and must prioritize research areas. String theory competes with other fields, such as particle physics, astrophysics, and materials science, for a share of these resources. Advances and discoveries in other fields can shift funding priorities.
- Public perception and political priorities: Public understanding and support for basic research, along with the political climate and governmental priorities, play a significant role. Periods of economic downturn or shifts in national priorities can lead to reduced funding for basic scientific research, including string theory.
- Peer review and evaluation processes: The rigorous peer review process used by funding agencies influences the allocation of grants. Proposals judged to be of high scientific merit and likely to produce significant results are more likely to receive funding.
Impact of Funding on String Theory Research
The level of funding directly impacts the progress and direction of string theory research in several ways:
- Number of researchers and research positions: Adequate funding enables the support of researchers, post-doctoral fellows, and graduate students, fostering the growth and development of the field.
- Availability of resources and infrastructure: Funding provides access to computational resources, experimental facilities (where relevant), and travel support for conferences and collaborations.
- Research focus and direction: Funding decisions can influence the direction of research. For example, funding agencies may prioritize projects with potential for near-term applications or those addressing fundamental questions with broader implications.
- International collaborations: Funding can support international collaborations, allowing researchers from different countries to work together on large-scale projects.
Public Perception and Misconceptions
String theory, a profoundly elegant yet incredibly complex attempt to unify all forces of nature, often suffers from significant misinterpretations in the public sphere. This stems from a confluence of factors, including the inherent difficulty of the subject matter, the limitations of popular science communication, and the influence of science fiction. Understanding these misconceptions is crucial for improving public engagement with this vital area of theoretical physics.
Common Misconceptions about String Theory
Several prevalent misunderstandings about string theory cloud public perception. These misconceptions often arise from oversimplification in popular media, misinterpretations of scientific concepts, or the influence of science fiction narratives. A clear understanding of these errors is essential for effective communication.
Misconception | Example | Origin Category |
---|---|---|
String theory is a fully proven theory. | “Scientists have confirmed string theory, explaining everything from the Big Bang to black holes.” | Simplification in popular media |
String theory is about tiny vibrating strings that make up everything. | “Everything is made of tiny vibrating strings, like musical notes creating a cosmic symphony.” | Misinterpretation of scientific concepts |
String theory predicts specific, easily testable outcomes. | “String theory’s predictions are readily verifiable through current experiments at the LHC.” | Simplification in popular media |
String theory is just a mathematical fantasy with no connection to reality. | “String theory is pure mathematics, detached from the physical world and lacking any experimental support.” | Misinterpretation of scientific concepts |
String theory solves all the problems of physics. | “String theory is the ‘Theory of Everything,’ resolving all mysteries of the universe.” | Influence of science fiction |
Reasons for Misunderstandings of String Theory
The complexity of string theory, requiring advanced mathematics and abstract concepts, contributes significantly to public misunderstanding. The lack of readily accessible explanations, often resorting to overly simplified analogies that can be misleading, exacerbates the problem. Metaphorical language, while helpful in some contexts, can also lead to misinterpretations if not carefully handled.Popular science media plays a critical role in shaping public perception.
While some documentaries and books offer valuable insights, others oversimplify or sensationalize the theory, contributing to misconceptions. For instance, the portrayal of string theory as a fully realized “Theory of Everything” is misleading, ignoring the ongoing challenges and debates within the field. Similarly, the focus on the “vibrating strings” aspect, without sufficient context about the higher-dimensional spaces and other intricate features, can create a distorted image.Science fiction, with its imaginative portrayals of parallel universes and advanced technologies, has both positive and negative impacts.
While it can stimulate interest in science, it often presents highly speculative and inaccurate depictions of string theory, fueling fantastical misconceptions. For example, many science fiction works portray easy manipulation of spacetime or travel between universes as direct consequences of string theory, which is far from the current scientific understanding.
Strategies for Improving Public Understanding of String Theory
Improving public understanding requires multifaceted strategies focusing on accessible communication and engaging content.
- Develop Interactive Educational Materials:
- Create interactive simulations and visualizations to illustrate complex concepts like extra dimensions or Calabi-Yau manifolds.
- Develop online courses and educational games that explain the fundamental ideas in a playful and engaging way.
- Challenge: Ensuring accuracy while maintaining accessibility and engaging design requires significant expertise in both physics and educational technology.
- Promote Clear and Accurate Popular Science Communication:
- Encourage scientists to actively participate in public outreach, emphasizing clarity and accuracy over sensationalism.
- Support the creation of high-quality popular science books, articles, and documentaries that present the theory responsibly.
- Challenge: Balancing accuracy with the need for accessible language requires careful consideration and skilled communication.
- Integrate String Theory into K-12 Education (Gradually and Appropriately):
- Introduce age-appropriate simplified concepts related to fundamental forces and the quest for unification into school curricula.
- Develop teaching materials that use analogies and storytelling to make abstract ideas more understandable.
- Challenge: Finding the right balance between simplified explanations and avoiding misinterpretations requires careful curriculum development and teacher training.
Existing public outreach initiatives show varying degrees of success. Some interactive exhibits in science museums have proven effective, while others have failed to engage the public due to overly technical presentations or a lack of interactive elements. Successful approaches typically involve analogies and visualizations that connect abstract concepts to everyday experiences. For example, explaining extra dimensions using the analogy of an ant crawling on a garden hose, where the ant only perceives one dimension (the length of the hose) while the hose itself exists in three dimensions, can be effective.
Ethical Implications of Communicating String Theory
Communicating complex scientific theories ethically requires a delicate balance. Oversimplification can lead to inaccuracies and misunderstandings, while overly technical explanations can alienate the public. The risk of misrepresentation, whether intentional or unintentional, can damage public trust in science. Responsible communication requires careful consideration of the target audience and a commitment to accuracy and transparency.
The Future Directions of String Theory Research
/GettyImages-499157257-56fd75565f9b586195c9dddc.jpg)
String theory, despite its challenges, remains a vibrant area of theoretical physics, offering a potential pathway to unifying gravity with quantum mechanics. Its future hinges on addressing fundamental open questions and developing innovative research strategies. The following sections Artikel key areas requiring focused attention.
Major Open Questions and Challenges
The landscape of string theory presents a formidable hurdle. The vast number of possible solutions, or vacua, makes it difficult to identify the one that corresponds to our universe. This landscape problem is not merely a philosophical concern; it significantly impacts the theory’s predictive power.
The Landscape Problem
The string theory landscape is estimated to contain a number of possible vacua exceeding 10 500, each representing a potentially different universe with distinct physical laws. This enormous number stems from the diverse ways in which extra spatial dimensions can be compactified. For instance, different Calabi-Yau manifolds, complex six-dimensional spaces used in compactification, lead to vastly different low-energy effective theories.
Specific models, like those based on type IIB string theory with fluxes, have been shown to generate incredibly large numbers of solutions. The challenge lies in developing criteria to select a physically relevant vacuum from this overwhelming multitude, potentially incorporating anthropic principles or novel physical constraints. This requires a deeper understanding of the underlying mathematical structure governing the landscape and the development of new tools to navigate it effectively.
The sheer scale makes exhaustive exploration computationally infeasible.
Experimental Verification
Currently, directly testing string theory predictions is extremely challenging due to the energy scales involved. The theory predicts phenomena that occur at energy levels far beyond the reach of current or foreseeable experiments. However, indirect evidence could be obtained through cosmological observations or the discovery of new particles at high-energy colliders.
Experimental Verification: Proposed Experiments and Potential Outcomes
Experiment/Observation | Feasibility (Near-term/Long-term) | Potential Outcome (Supporting/Refuting aspects) | Specific String Theory Prediction Tested |
---|---|---|---|
High-energy collider experiments (e.g., future colliders exceeding LHC energy) | Long-term | Could reveal supersymmetric particles or other exotic particles predicted by specific string models. Absence would constrain or refute certain models. | Existence of supersymmetric particles, specific particle masses and interactions |
Cosmological observations (e.g., precise measurements of the cosmic microwave background, large-scale structure surveys) | Near-term/Long-term | Could reveal evidence for extra dimensions through subtle effects on gravitational interactions or anisotropies in the CMB. | Specific features of the early universe, signatures of string compactifications |
Gravitational wave detection (e.g., detection of gravitational waves from primordial black holes) | Near-term/Long-term | Could reveal information about black hole properties that could support or refute aspects of string theory’s description of black holes. | Black hole entropy, information paradox resolution mechanisms |
Mathematical Rigor
A significant challenge lies in developing a fully non-perturbative formulation of string theory. Current approaches rely heavily on perturbative expansions, which are approximations valid only under certain conditions. A non-perturbative formulation would provide a more complete and rigorous understanding of the theory’s fundamental principles, potentially resolving issues like the landscape problem. Another challenge is the lack of a complete understanding of string field theory, a framework aimed at describing non-perturbative effects.
Moreover, rigorous mathematical proofs for many key results, especially those related to string dualities, are still lacking. Progress in areas such as algebraic geometry, topology, and representation theory will be crucial.
Potential Avenues for Future Research and Development
Addressing the challenges Artikeld above requires exploring several promising avenues of research.
AdS/CFT Correspondence
The AdS/CFT correspondence, a duality between a gravitational theory in anti-de Sitter (AdS) spacetime and a conformal field theory (CFT) in one fewer dimension, offers a powerful tool for studying quantum gravity. Open problems include a deeper understanding of the correspondence beyond the large N limit (where N is the number of colors in the gauge theory), exploring quantum gravity in more general curved spacetimes, and extending applications to condensed matter physics.
For instance, research could focus on using AdS/CFT to model strongly coupled systems in condensed matter physics, such as high-temperature superconductors.
String Phenomenology
String phenomenology aims to connect string theory to observable phenomena. Specific models, like those based on heterotic string theory or type II string theory with D-branes, attempt to derive the Standard Model of particle physics from string theory. These models often predict new particles or interactions that could be tested experimentally. For example, some models predict the existence of extra gauge bosons or specific supersymmetric particles with measurable properties.
The development of more realistic models that accurately reproduce the Standard Model and its parameters remains a significant challenge.
Development of New Mathematical Tools
The development of new mathematical tools is crucial for advancing string theory. Areas like higher category theory, homological algebra, and non-commutative geometry offer promising avenues for tackling the mathematical complexities of the theory. These tools could help in formulating a non-perturbative description of string theory, resolving issues related to the landscape problem, and developing a more complete understanding of string dualities.
The development of more sophisticated computational techniques, such as machine learning algorithms, is also essential for analyzing complex string theory calculations and exploring the vast landscape of string vacua.
Possible Breakthroughs and Overcoming Current Limitations
Significant progress could result from breakthroughs in several key areas.
Unification with Other Fundamental Theories
Unifying string theory with other fundamental theories, such as loop quantum gravity or quantum field theory, is a long-term goal. This could involve developing a more general framework encompassing both approaches or discovering unexpected connections between them. For example, the search for a unified theory might involve exploring connections between string theory and twistor theory, a mathematical framework used in quantum field theory.
Successful unification would provide a more complete and consistent description of the fundamental forces and particles in the universe.
Development of a Complete Non-Perturbative Formulation, Is string theory dead
Developing a complete non-perturbative formulation of string theory is a major challenge. Approaches such as matrix models, topological string theory, and string field theory offer potential pathways towards this goal. A non-perturbative formulation would provide a more complete and rigorous understanding of the theory’s fundamental principles and would be essential for addressing the landscape problem and making more precise predictions.
Novel Computational Techniques
Employing novel computational techniques, such as machine learning and artificial intelligence, could significantly accelerate progress in string theory. These techniques could be used to explore the string theory landscape more efficiently, analyze complex calculations, and identify patterns that might not be apparent through traditional methods. For example, machine learning algorithms could be used to classify string vacua based on their physical properties or to identify promising avenues for research based on large datasets of string theory calculations.
Illustrative Example
This section presents a conceptual diagram illustrating the concept of branes in string theory, specifically focusing on how our four-dimensional universe might be embedded within a higher-dimensional spacetime. The diagram simplifies a complex mathematical construct to provide a visual aid for understanding a key aspect of string theory.This diagram depicts a simplified model of brane cosmology, showing our universe as a three-dimensional brane embedded within a higher-dimensional spacetime, often referred to as the “bulk.” The limitations of representing higher dimensions visually necessitate simplification, but the diagram aims to convey the fundamental concept.
Brane Embedding in Higher Dimensions
The diagram should be a two-dimensional representation of a higher-dimensional space. Imagine a flat, rectangular surface representing a three-dimensional spatial slice of our universe (a 3-brane). This 3-brane is depicted as a relatively small rectangle within a larger, encompassing space. This larger space represents the higher-dimensional bulk, which could have, for instance, ten or eleven dimensions in some string theory models.
The extra dimensions are not explicitly shown as separate axes, as this is graphically challenging; their presence is implied by the embedding of the 3-brane within the larger space. The 3-brane is not simply floating within the bulk; it is intrinsically linked to it, much like a sheet of paper is part of the three-dimensional space it occupies.The bulk space itself can be represented as a larger rectangle, possibly with subtly different shading or texture to distinguish it from the 3-brane.
This difference could represent the different physical laws or properties of the bulk compared to those within our 3-brane universe. Gravity, for instance, might be depicted as a field that extends beyond the 3-brane into the bulk, explaining why gravity appears weaker than other forces in our universe. This weakness could be visually suggested by a progressively fading representation of the gravitational field as it extends away from the 3-brane.Furthermore, one could consider adding small, simplified representations of other branes within the bulk, potentially interacting with our 3-brane.
These other branes could be represented as smaller, differently colored rectangles, illustrating the potential for interactions and the multiverse concept within some brane-world scenarios. These interactions could be represented symbolically, perhaps with faint lines connecting our brane to others. The distances between branes should be represented as significantly larger than the dimensions of the branes themselves, to emphasize the vastness of the bulk space.
Finally, the diagram should include a clear legend explaining the various elements depicted, such as the 3-brane representing our universe, the bulk space, and any additional branes included. The legend should also clearly indicate that the diagram is a simplified representation and does not accurately reflect the complex mathematical nature of the concept.
Illustrative Example
This section details a hypothetical experiment designed to test a specific prediction of string theory: the existence of Kaluza-Klein gravitons arising from compactified extra dimensions. This prediction, while not directly observable with current technology, provides a concrete target for future experimental efforts. The experiment focuses on detecting the characteristic energy signature of these gravitons, a signature predicted to be detectable in high-energy particle collisions.
Experimental Setup
The proposed experiment utilizes a significantly enhanced version of the Large Hadron Collider (LHC), designated the “Kaluza-Klein Collider” (KKC). This KKC would boast a substantially higher collision energy, reaching several orders of magnitude beyond the LHC’s capabilities, potentially achieving energies in the TeV to PeV range. It would also incorporate highly advanced detectors designed to identify the unique characteristics of Kaluza-Klein gravitons.
The KKC would necessitate a larger ring circumference, requiring substantial engineering and logistical challenges. Crucially, the KKC’s detectors would need unprecedented sensitivity to detect low-energy decay products resulting from the extremely high-energy collisions.The environmental considerations for the KKC are critical. The experiment requires an ultra-high vacuum environment, minimizing particle scattering. Precise temperature control is essential to maintain the stability of the superconducting magnets and the detectors.
Electromagnetic shielding would be implemented to reduce external interference. The facility itself would need to be located far underground, shielded from cosmic rays.
Experimental Methodology
Data acquisition would involve recording all particle interactions within the KKC. The detectors would focus on identifying low-energy decay products from the high-energy collisions, searching for patterns consistent with the decay of Kaluza-Klein gravitons. This would involve sophisticated algorithms designed to differentiate between the decay signatures of Kaluza-Klein gravitons and other background processes.Data analysis would rely on advanced statistical techniques to identify subtle patterns in the data.
The significance of any observed signal would be assessed using rigorous statistical tests, comparing the observed data to predictions from both the string theory model and standard model backgrounds. This will involve sophisticated machine learning algorithms to filter out noise and identify patterns consistent with Kaluza-Klein graviton decay.Control experiments would be essential to rule out other explanations for any observed signals.
This includes calibrating the detectors using known particle interactions, running simulations to model background processes, and comparing results across different collision energies and detector configurations.
Expected Results
Positive results would manifest as an excess of low-energy events with specific energy and angular distributions consistent with the predicted decay products of Kaluza-Klein gravitons. For example, we might expect to observe a statistically significant excess of events with specific invariant masses, corresponding to the predicted masses of Kaluza-Klein gravitons. These events should also exhibit specific angular correlations, absent in background processes.Negative results, the absence of any such excess, would suggest that the specific string theory prediction under investigation is incorrect, or at least requires modification.
This would not necessarily invalidate string theory as a whole, but it would necessitate a re-evaluation of the specific model used to make the prediction.
Potential Sources of Error
Source of Error | Description | Mitigation Strategy |
---|---|---|
Background noise | Interference from cosmic rays, detector noise, and other background processes | Advanced filtering techniques, sophisticated data analysis algorithms, improved shielding |
Calibration inaccuracies | Imperfect calibration of the detectors and their associated electronics | Regular calibration checks, cross-calibration methods, redundancy in detector systems |
Systematic errors | Bias introduced by the experimental setup, data acquisition, or analysis methods | Careful design of the experiment, rigorous testing and validation of the analysis methods, multiple independent analysis teams |
Theoretical uncertainties | Uncertainties in the theoretical predictions for Kaluza-Klein graviton properties | Refining theoretical calculations, exploring different string theory models |
Summary
The proposed Kaluza-Klein Collider experiment aims to directly test a specific prediction of string theory concerning the existence of Kaluza-Klein gravitons arising from compactified extra dimensions. The experiment requires a significant advancement in accelerator technology and detector sensitivity, but its potential payoff is enormous. A positive result would provide compelling evidence for extra spatial dimensions and significantly advance our understanding of quantum gravity.
A negative result, while not disproving string theory, would nonetheless provide valuable insights into its limitations and guide future theoretical developments. This experiment represents a crucial step in bridging the gap between theoretical predictions and experimental verification in the field of string theory.
FAQ Resource
What are some common misunderstandings about string theory?
Many think string theory is “just a theory” implying it’s not well-supported. It’s a scientific theory, a well-developed framework with mathematical consistency, though experimental verification is a huge challenge. Another misconception is that it’s a single, unified theory. Actually, it encompasses many different versions and approaches.
Why is it so hard to test string theory experimentally?
Simply put, the energy scales required to directly observe string-scale phenomena are far beyond our current technological capabilities. Plus, distinguishing string theory’s predicted signatures from background noise is extremely difficult.
Are there any alternative theories to string theory?
Yes! Loop quantum gravity, causal set theory, and asymptotic safety are a few examples of competing theories attempting to reconcile general relativity and quantum mechanics.
What is the “string theory landscape”?
The string theory landscape refers to the vast number of possible solutions or “vacua” within the theory. This makes it difficult to make specific, testable predictions.
What is the role of supersymmetry in string theory?
Supersymmetry is a key ingredient in many string theory models, postulating a symmetry between bosons and fermions. However, experimental evidence for supersymmetry remains elusive.